电力电子器件应用指南
电力电子技术中的电流传感器选型指南

电力电子技术中的电流传感器选型指南电力电子技术中,电流传感器的选型对于系统性能和稳定性至关重要。
正确选择适合的电流传感器可以确保电路的可靠性和效率,同时也可以提高工作效果和安全性。
本指南将介绍电力电子技术中电流传感器的选型原则和注意事项。
一、电流传感器的作用和分类电流传感器在电力电子技术中被广泛应用,主要用于测量和监控电力系统中的电流。
根据其原理和测量方式,电流传感器可以分为多种类型,包括霍尔效应传感器、电流互感器和电阻分压式传感器等。
不同类型的电流传感器适用于不同的应用场景。
二、选型原则和要考虑的因素1. 电流范围:选择电流传感器时,首先要考虑的是测量范围。
根据实际应用需求,确定所需的电流测量范围,并选择合适的电流传感器。
2. 精度和灵敏度:电流传感器的精度和灵敏度直接影响到测量结果的准确性。
在选型时,需要根据实际需求评估其精度和灵敏度指标,并选择性能合适的传感器。
3. 响应时间:对于某些需要实时监测的应用,电流传感器的响应时间非常重要。
根据要求的监测速度和实时性,选择具有较短响应时间的传感器。
4. 绝缘性能:在电力电子技术中,绝缘性能很重要,特别是在高压电力系统中的应用。
选择具有良好绝缘性能的电流传感器,以确保系统的安全性和稳定性。
5. 温度特性:电流传感器的性能在不同温度下可能变化,因此需要考虑其温度特性。
根据实际应用环境和工作温度范围,选择适合的电流传感器。
三、常见问题及解决方案1. 大电流的测量:对于需测量大电流的应用,可以选择低阻抗的电流传感器,以避免因传感器本身的电阻损耗而导致的测量误差。
2. 系统故障和电磁干扰:在电力电子技术中,电流传感器容易受到系统故障和电磁干扰的影响。
为了提高测量的准确性和稳定性,可以选择具有抗干扰能力较强的传感器,并采取合适的屏蔽和滤波措施。
3. 安装和连接:在安装和连接电流传感器时,应遵循制造商提供的安装指导,并确保连接可靠和稳定,以避免因不良连接而导致的测量误差。
IPM(智能功率模块)应用手册
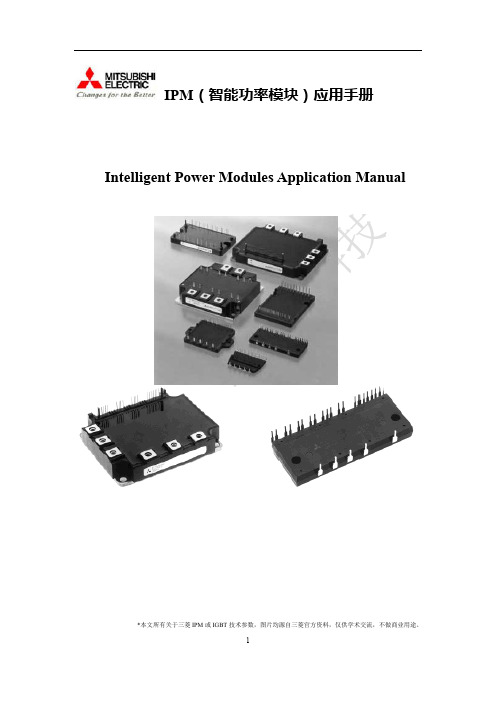
IPM (智能功率模块)应用手册Intelligent Power Modules Application Manual*本文所有关于三菱IPM 或IGBT 技术参数,图片均源自三菱官方资料,仅供学术交流,不做商业用途。
目录1.引言 (5)2.IPM(智能功率模块)的一般认识 (5)2.1.功率电路之设计 (5)a.关断浪涌电压b.续流二极管恢复浪涌c.接地回路d.减小功率电路之电感2.2吸收电路之设计 (6)a.吸收电路的类型b.吸收电感的作用c.母线电感的作用d.功率电路和吸收电路设计的建议2.3功耗设计 (8)a.功耗的估算b.VVVF变频器功耗的计算c.平均结温的估算d.瞬态温升的估算e.散热器之安装3.IPM的前身-IGBT模块的使用 (11)3.1. IGBT模块的结构和工作原理 (11)3.2.IGBT模块的额定值和特性 (11)a.最大额定值b.电气特性c.热阻3.3.特性曲线 (12)a.输出特性b.饱和特性c.开关特性3.4栅极驱动及模块的保护 (13)a.驱动电压b.串联栅极电阻(R G)c.栅极驱动所须功率要求d.栅极驱动布线注意e.dv/dt保护f.短路保护4.IPM智能功率模块的使用 (16)4.1.IPM的结构 (16)a.多层环氧树脂工艺b.铜箔直接铸接工艺c.IPM的优点4.2.IPM额定值和特性 (19)a.最大额定值b.热阻c.电气特性d.推荐工作条件4.3.安全工作区 (21)a.开关安全工作区b.短路安全工作区4.4.IPM的保护功能 (21)a.自保护特性b.控制电源的欠压锁定(UV)c.过热保护(OT)d.过流保护(OC)e.短路保护(SC)4.5.IPM的选用 (24)4.6.控制电路电源a.IPM的控制电源功率消耗b.布线指南c.电路结构4.7.IPM接口电路 (25)a.接口电路要求b.布线c.内部输入输出电路d. 连接接口电路e. 死区时间(T d )f.故障信号FO 输出的使用 g. IPM 的一般应用h.一般变频系统的结构MCU1. 引言:把MOS管技术引入功率半导体器件的思想开创了革命性的器件:绝缘栅双极晶体管IGBT。
高压栅极驱动 ic 自举电路的设计与应用指南(

一、概述高压栅极驱动IC自举电路是一种常用于驱动MOSFET等功率器件的电路,其设计与应用对于提高系统的性能和稳定性具有重要意义。
本文将从基本原理、设计要点和实际应用等方面,对高压栅极驱动IC自举电路进行全面系统的介绍和分析,旨在为工程师和研究人员提供一份全面且实用的指南。
二、基本原理1. 高压栅极驱动IC自举电路的概念高压栅极驱动IC(Integrated Circuit)自举电路是一种能够产生驱动信号所需的高压电源的电路,通常用于驱动功率开关器件(如MOSFET、IGBT等)。
2. 自举电路的工作原理自举电路通过外部电容器储存电荷,在需要驱动时将这部分电荷释放,从而形成高压供电。
该电路能够有效地提供驱动信号所需的高电压,同时具有简单、高效等特点。
三、设计要点1. 电容器的选择在设计高压栅极驱动IC自举电路时,电容器的特性对电路的性能具有重要影响。
电容器的选择应考虑其容量、工作电压和频率特性等。
2. 电源管理电路自举电路需要有稳定可靠的电源管理电路,来保证其供电过程的稳定性和可靠性。
在设计时应选用合适的稳压器、电源管理IC等器件。
3. 驱动信号的匹配高压栅极驱动IC自举电路应能够有效地匹配待驱动器件的输入电压和电流要求,以确保系统的性能和稳定性。
四、实际应用1. 在功率电子系统中的应用高压栅极驱动IC自举电路广泛应用于各种功率电子系统中,如电源逆变器、电机驱动器、变流器等。
2. 在新能源领域的应用随着新能源技术的快速发展,高压栅极驱动IC自举电路在太阳能、风能等领域得到了广泛的应用,为新能源系统的高效工作提供了重要支持。
五、总结高压栅极驱动IC自举电路作为一种常见的功率器件驱动方案,在现代电子系统中具有重要的应用价值。
本文通过对其基本原理、设计要点和实际应用进行了全面介绍,旨在帮助读者更好地了解和应用这一技术,并在实际工程中取得更好的效果。
文章的篇幅可能不足3000字,需要根据实际情况继续扩展内容。
三极管手册介绍

三极管手册介绍
三极管,也称为晶体三极管,是一种常用的电子器件,被广泛应用于电子电路中。
它由三个区域相互夹杂的半导体材料构成,通常被标记为E(发射极)、B(基极)和C(集电极)。
三极管是一种双极型晶体管,其主要特点是能够控制电流放大倍数。
通过控制基极电流,可以控制集电极电流的放大倍数。
因此,三极管广泛用于放大、开关、电子开关、振荡器等电路中。
三极管手册是一本关于三极管的详细介绍和应用指南。
该手册通常包括以下内容:
1. 三极管的基础知识:介绍三极管的结构、工作原理和基本参数。
包括器件标记和引脚配置,以及不同类型的三极管(如NPN型和PNP型)。
2. 三极管的电路应用:包括放大电路、开关电路、电源电路、振荡电路和稳压电路等。
每个电路应用都会介绍其原理、设计方法、常用电路图和计算公式。
3. 三极管的参数与曲线特性:包括直流参数(如最大集电流、最大功耗、最大电压等)和交流参数(如频率响应、增益、噪声系数等)。
手册中通常会给出参数的定义、测量方法和典型数值。
4. 三极管的选型与应用:介绍如何根据特定的应用需求选择合
适的三极管。
包括选择参数的考虑因素、常用的选型指南和技术手段。
5. 三极管的常见故障排除:介绍三极管常见的故障原因及排除方法。
包括电压过高、电流过大、温度过高等故障的检测和解决方法。
综上所述,三极管手册是一本提供关于三极管结构、工作原理、电路应用、参数与曲线特性、选型与应用和故障排除等方面知识的参考指南,旨在帮助工程师和电子爱好者更好地理解和应用三极管。
电子电工技术的实际应用指南及案例分析
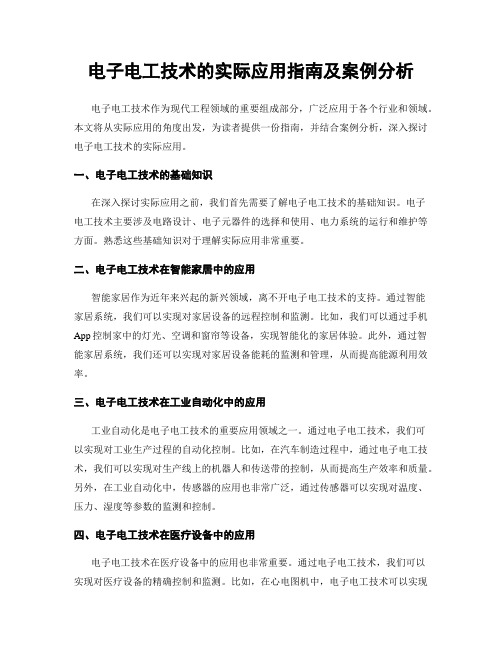
电子电工技术的实际应用指南及案例分析电子电工技术作为现代工程领域的重要组成部分,广泛应用于各个行业和领域。
本文将从实际应用的角度出发,为读者提供一份指南,并结合案例分析,深入探讨电子电工技术的实际应用。
一、电子电工技术的基础知识在深入探讨实际应用之前,我们首先需要了解电子电工技术的基础知识。
电子电工技术主要涉及电路设计、电子元器件的选择和使用、电力系统的运行和维护等方面。
熟悉这些基础知识对于理解实际应用非常重要。
二、电子电工技术在智能家居中的应用智能家居作为近年来兴起的新兴领域,离不开电子电工技术的支持。
通过智能家居系统,我们可以实现对家居设备的远程控制和监测。
比如,我们可以通过手机App控制家中的灯光、空调和窗帘等设备,实现智能化的家居体验。
此外,通过智能家居系统,我们还可以实现对家居设备能耗的监测和管理,从而提高能源利用效率。
三、电子电工技术在工业自动化中的应用工业自动化是电子电工技术的重要应用领域之一。
通过电子电工技术,我们可以实现对工业生产过程的自动化控制。
比如,在汽车制造过程中,通过电子电工技术,我们可以实现对生产线上的机器人和传送带的控制,从而提高生产效率和质量。
另外,在工业自动化中,传感器的应用也非常广泛,通过传感器可以实现对温度、压力、湿度等参数的监测和控制。
四、电子电工技术在医疗设备中的应用电子电工技术在医疗设备中的应用也非常重要。
通过电子电工技术,我们可以实现对医疗设备的精确控制和监测。
比如,在心电图机中,电子电工技术可以实现对心电信号的放大和滤波,从而提高心电图的准确性。
另外,在医疗设备中,电子电工技术还可以实现对医疗设备的远程监测和管理,从而提高医疗设备的使用效率。
五、电子电工技术在能源领域中的应用能源领域是电子电工技术的另一个重要应用领域。
通过电子电工技术,我们可以实现对能源的高效利用和管理。
比如,在太阳能发电系统中,电子电工技术可以实现对太阳能电池板的光电转换和电能输出的控制。
电力电子技术中的谐振变换器电阻选型指南
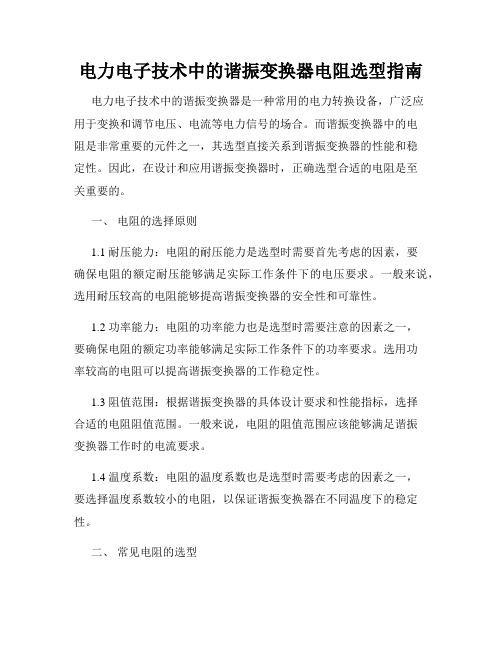
电力电子技术中的谐振变换器电阻选型指南电力电子技术中的谐振变换器是一种常用的电力转换设备,广泛应用于变换和调节电压、电流等电力信号的场合。
而谐振变换器中的电阻是非常重要的元件之一,其选型直接关系到谐振变换器的性能和稳定性。
因此,在设计和应用谐振变换器时,正确选型合适的电阻是至关重要的。
一、电阻的选择原则1.1 耐压能力:电阻的耐压能力是选型时需要首先考虑的因素,要确保电阻的额定耐压能够满足实际工作条件下的电压要求。
一般来说,选用耐压较高的电阻能够提高谐振变换器的安全性和可靠性。
1.2 功率能力:电阻的功率能力也是选型时需要注意的因素之一,要确保电阻的额定功率能够满足实际工作条件下的功率要求。
选用功率较高的电阻可以提高谐振变换器的工作稳定性。
1.3 阻值范围:根据谐振变换器的具体设计要求和性能指标,选择合适的电阻阻值范围。
一般来说,电阻的阻值范围应该能够满足谐振变换器工作时的电流要求。
1.4 温度系数:电阻的温度系数也是选型时需要考虑的因素之一,要选择温度系数较小的电阻,以保证谐振变换器在不同温度下的稳定性。
二、常见电阻的选型2.1 固定电阻:固定电阻是谐振变换器常用的电阻类型,分为碳膜电阻、金属膜电阻、瓷体电阻等。
根据实际需求选用合适的固定电阻类型,并根据电阻的耐压能力、功率能力、阻值范围和温度系数等参数进行选型。
2.2 动态电阻:动态电阻也是谐振变换器中常用的电阻类型,它具有根据电流和电压变化调节阻值的特性,可以实现对谐振变换器的动态监测和控制。
根据动态电阻的特性和性能参数进行选型,确保其能够满足谐振变换器的工作要求。
2.3 光敏电阻:光敏电阻是一种特殊的电阻器件,其阻值随光照强度变化而变化。
在一些特殊的谐振变换器设计中,可以选用光敏电阻实现对谐振变换器的光照监测和控制。
三、电阻的应用注意事项3.1 电阻的布局:在设计谐振变换器电路时,合理布局电阻的位置是非常重要的。
要尽量将电阻与其他元件隔离,避免互相干扰和影响,确保谐振变换器的稳定性和可靠性。
IGBT数据手册
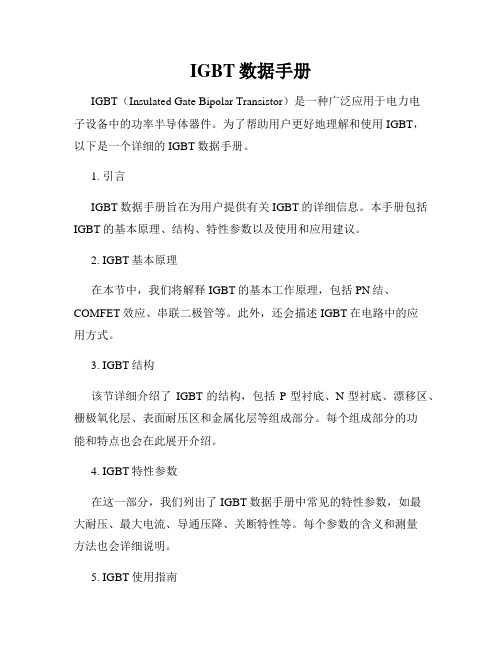
IGBT数据手册IGBT(Insulated Gate Bipolar Transistor)是一种广泛应用于电力电子设备中的功率半导体器件。
为了帮助用户更好地理解和使用IGBT,以下是一个详细的IGBT数据手册。
1. 引言IGBT数据手册旨在为用户提供有关IGBT的详细信息。
本手册包括IGBT的基本原理、结构、特性参数以及使用和应用建议。
2. IGBT基本原理在本节中,我们将解释IGBT的基本工作原理,包括PN结、COMFET效应、串联二极管等。
此外,还会描述IGBT在电路中的应用方式。
3. IGBT结构该节详细介绍了IGBT的结构,包括P型衬底、N型衬底、漂移区、栅极氧化层、表面耐压区和金属化层等组成部分。
每个组成部分的功能和特点也会在此展开介绍。
4. IGBT特性参数在这一部分,我们列出了IGBT数据手册中常见的特性参数,如最大耐压、最大电流、导通压降、关断特性等。
每个参数的含义和测量方法也会详细说明。
5. IGBT使用指南本节将提供有关IGBT的使用和应用建议。
包括IGBT的散热、驱动电路设计、保护电路设置以及注意事项等方面的内容。
这些指南将帮助用户更好地应用和操作IGBT。
6. IGBT应用示例为了更好地理解IGBT的实际应用,我们将在本节中提供一些IGBT在不同领域的应用示例,如交流调速、电力变换器、电机驱动等。
每个示例将简要介绍应用场景和IGBT在其中的作用。
7. 总结在最后一节中,我们将对整个IGBT数据手册进行总结,并再次强调IGBT的重要性和广泛应用领域。
如果读者有任何疑问或需要进一步了解,也可以参阅其他相关资料或直接联系我们的技术支持团队。
结论:通过本IGBT数据手册,相信用户能够更好地了解和使用IGBT。
同时,本手册也提供了IGBT的基本原理、结构、特性参数以及使用和应用建议等方面的详细信息。
希望本手册对用户有所帮助,并欢迎提供反馈和建议,以便我们进一步改进和完善IGBT数据手册。
电力电子技术中的谐振变换器电容选型指南
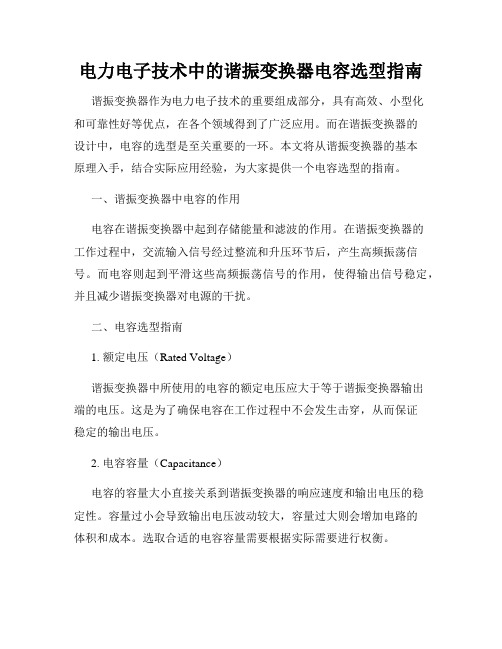
电力电子技术中的谐振变换器电容选型指南谐振变换器作为电力电子技术的重要组成部分,具有高效、小型化和可靠性好等优点,在各个领域得到了广泛应用。
而在谐振变换器的设计中,电容的选型是至关重要的一环。
本文将从谐振变换器的基本原理入手,结合实际应用经验,为大家提供一个电容选型的指南。
一、谐振变换器中电容的作用电容在谐振变换器中起到存储能量和滤波的作用。
在谐振变换器的工作过程中,交流输入信号经过整流和升压环节后,产生高频振荡信号。
而电容则起到平滑这些高频振荡信号的作用,使得输出信号稳定,并且减少谐振变换器对电源的干扰。
二、电容选型指南1. 额定电压(Rated Voltage)谐振变换器中所使用的电容的额定电压应大于等于谐振变换器输出端的电压。
这是为了确保电容在工作过程中不会发生击穿,从而保证稳定的输出电压。
2. 电容容量(Capacitance)电容的容量大小直接关系到谐振变换器的响应速度和输出电压的稳定性。
容量过小会导致输出电压波动较大,容量过大则会增加电路的体积和成本。
选取合适的电容容量需要根据实际需要进行权衡。
对于高频谐振变换器,一般采用电容的容值较小,以便提高响应速度和效率。
而对于低频谐振变换器,电容的容值可以适当增大,以保证输出电压的稳定性。
3. 电容ESR(Equivalent Series Resistance)电容的ESR是指实际电容器中所包含的电阻和等效电感。
ESR对谐振变换器的效率和稳定性有重要影响。
ESR过大会导致能量损耗较大,温升过高;ESR过小则容易引起共振和不稳定的问题。
因此,在电容选型中,需要注意选择具有较低ESR的电容,以提高谐振变换器的效率和稳定性。
4. 锁相电容(Snubber Capacitor)锁相电容也是谐振变换器中常用的一种电容类型。
它的作用是减小开关管的开关损耗和谐振电压幅值,从而提高谐振变换器的性能。
锁相电容的选取需要结合具体的设计需求和系统特点,一般可以通过仿真和实验来确定合适的容值。
电力电子技术中的电容选型及容值计算指南

电力电子技术中的电容选型及容值计算指南在电力电子技术中,电容是一种重要的元件,广泛应用于电源、逆变器、变频器等电路中。
正确选择和计算电容的容值,对于电路性能和稳定性非常关键。
本篇文章将介绍电力电子技术中电容的选型原则以及容值计算的指导方法。
一. 电容选型原则1. 工作电压 (Rated Voltage)在选择电容时,需要考虑电路中的最大工作电压。
工作电压应大于或等于电路中最大电压幅值的峰-峰值。
如果电容的工作电压过低,则容易导致电容击穿,从而引发故障。
2. 脉冲功率 (Pulse Power)对于一些需要传输脉冲功率的电路,比如在电动机驱动器中,电容必须具有足够大的脉冲功率承受能力。
因此,在选型时需要查阅电容的数据手册,确保其能够满足所需的脉冲功率要求。
3. 电容损耗 (Dielectric Losses)电容的损耗正比于工作频率和电容的介电损耗因子。
在高频应用中,为了减小能效损耗,应选择具有较低损耗因子的电容。
同时还需要考虑电容的温升和工作寿命。
4. 尺寸和体积 (Size and Volume)尺寸和体积是电容选型时需要考虑的另一个重要因素。
一般来说,选择具有更高介电常数的电容,可以减小其体积。
同时还需要考虑电容的容量,以满足电路中的电能存储需求。
二. 电容容值计算方法电容的容值选择取决于电路的需求和设计参数。
以下是一些电容容值计算的常用方法:1. 低通滤波器 (Low-Pass Filters)在低通滤波器中,电容的选择取决于滤波器的截止频率以及负载阻抗。
一般来说,电容的容值可以通过以下公式计算:C = 1 / (2πfR)其中,C为所需电容的容值,f为截止频率,R为负载阻抗。
2. 直流链接和绕组 (DC Link and Windings)对于直流链接和绕组,电容的容值需要根据电压涟漪和电流涟漪来选择。
一般来说,电容的容值可以通过以下公式计算:C = ΔI / (ΔV × f)其中,C为所需电容的容值,ΔI为电流涟漪的最大值,ΔV为电压涟漪的最大值,f为涟漪频率。
晶闸管(可控硅)应用指南
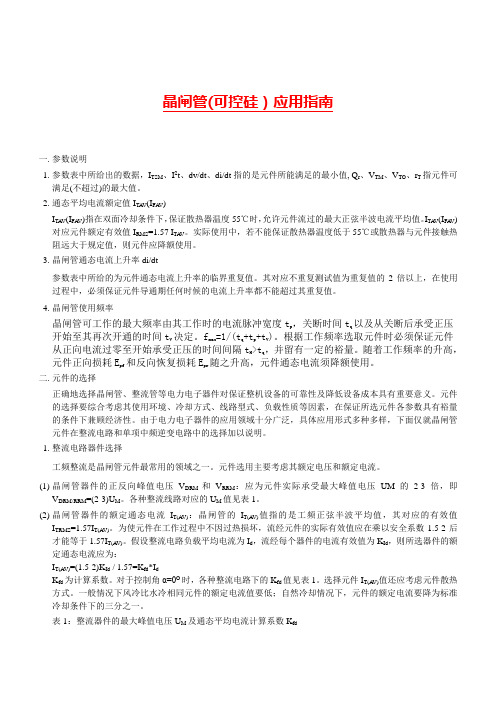
晶闸管(可控硅)应用指南一.参数说明1.参数表中所给出的数据,I TSM、I2t、dv/dt、di/dt指的是元件所能满足的最小值, Q r、V TM、V TO、r T指元件可满足(不超过)的最大值。
2.通态平均电流额定值I TA V(I FA V)I TA V(I FA V)指在双面冷却条件下,保证散热器温度55℃时,允许元件流过的最大正弦半波电流平均值。
I TA V(I FAV)对应元件额定有效值I RMS=1.57 I TA V。
实际使用中,若不能保证散热器温度低于55℃或散热器与元件接触热阻远大于规定值,则元件应降额使用。
3.晶闸管通态电流上升率di/dt参数表中所给的为元件通态电流上升率的临界重复值。
其对应不重复测试值为重复值的2倍以上,在使用过程中,必须保证元件导通期任何时候的电流上升率都不能超过其重复值。
4.晶闸管使用频率晶闸管可工作的最大频率由其工作时的电流脉冲宽度tp ,关断时间tq以及从关断后承受正压开始至其再次开通的时间tV 决定。
fmax=1/(tq+tp+tV)。
根据工作频率选取元件时必须保证元件从正向电流过零至开始承受正压的时间间隔tH >tq,并留有一定的裕量。
随着工作频率的升高,元件正向损耗Epf 和反向恢复损耗Epr随之升高,元件通态电流须降额使用。
二.元件的选择正确地选择晶闸管、整流管等电力电子器件对保证整机设备的可靠性及降低设备成本具有重要意义。
元件的选择要综合考虑其使用环境、冷却方式、线路型式、负载性质等因素,在保证所选元件各参数具有裕量的条件下兼顾经济性。
由于电力电子器件的应用领域十分广泛,具体应用形式多种多样,下面仅就晶闸管元件在整流电路和单项中频逆变电路中的选择加以说明。
1.整流电路器件选择工频整流是晶闸管元件最常用的领域之一。
元件选用主要考虑其额定电压和额定电流。
(1)晶闸管器件的正反向峰值电压V DRM和V RRM:应为元件实际承受最大峰值电压UM的2-3倍,即V DRM/RRM=(2-3)U M。
IGBT 模块 应用指南-基本知识
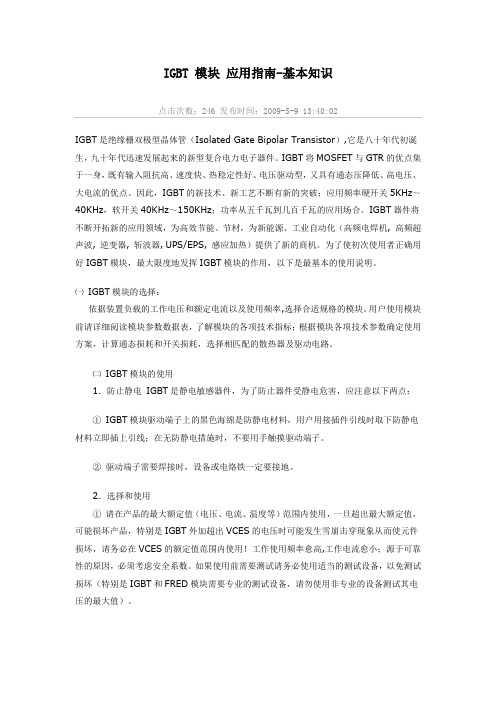
IGBT 模块应用指南-基本知识点击次数:246 发布时间:2009-5-9 13:40:02IGBT是绝缘栅双极型晶体管(Isolated Gate Bipolar Transistor),它是八十年代初诞生,九十年代迅速发展起来的新型复合电力电子器件。
IGBT将MOSFET与GTR的优点集于一身,既有输入阻抗高、速度快、热稳定性好、电压驱动型,又具有通态压降低、高电压、大电流的优点。
因此,IGBT的新技术、新工艺不断有新的突破;应用频率硬开关5KHz~40KHz,软开关40KHz~150KHz;功率从五千瓦到几百千瓦的应用场合。
IGBT器件将不断开拓新的应用领域,为高效节能、节材,为新能源、工业自动化(高频电焊机, 高频超声波, 逆变器, 斩波器, UPS/EPS, 感应加热)提供了新的商机。
为了使初次使用者正确用好IGBT模块,最大限度地发挥IGBT模块的作用,以下是最基本的使用说明。
㈠IGBT模块的选择:依据装置负载的工作电压和额定电流以及使用频率,选择合适规格的模块。
用户使用模块前请详细阅读模块参数数据表,了解模块的各项技术指标;根据模块各项技术参数确定使用方案,计算通态损耗和开关损耗,选择相匹配的散热器及驱动电路。
㈡IGBT模块的使用1.防止静电IGBT是静电敏感器件,为了防止器件受静电危害,应注意以下两点:①IGBT模块驱动端子上的黑色海绵是防静电材料,用户用接插件引线时取下防静电材料立即插上引线;在无防静电措施时,不要用手触摸驱动端子。
②驱动端子需要焊接时,设备或电烙铁一定要接地。
2.选择和使用①请在产品的最大额定值(电压、电流、温度等)范围内使用,一旦超出最大额定值,可能损坏产品,特别是IGBT外加超出VCES的电压时可能发生雪崩击穿现象从而使元件损坏,请务必在VCES的额定值范围内使用!工作使用频率愈高,工作电流愈小;源于可靠性的原因,必须考虑安全系数。
如果使用前需要测试请务必使用适当的测试设备,以免测试损坏(特别是IGBT和FRED模块需要专业的测试设备,请勿使用非专业的设备测试其电压的最大值)。
功率集成器件指南模板
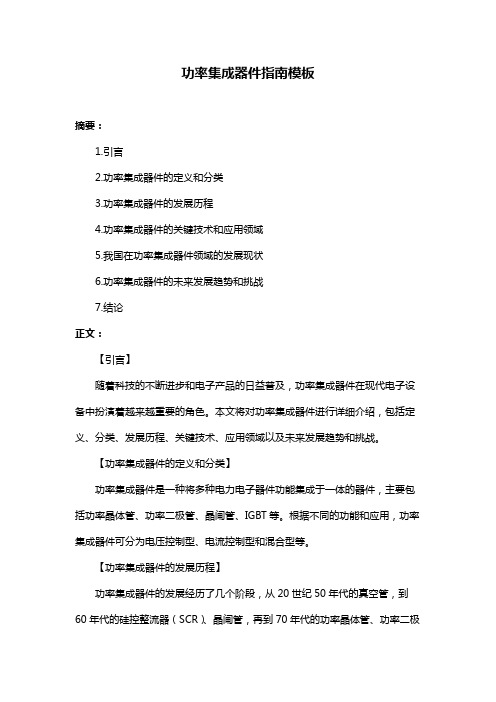
功率集成器件指南模板摘要:1.引言2.功率集成器件的定义和分类3.功率集成器件的发展历程4.功率集成器件的关键技术和应用领域5.我国在功率集成器件领域的发展现状6.功率集成器件的未来发展趋势和挑战7.结论正文:【引言】随着科技的不断进步和电子产品的日益普及,功率集成器件在现代电子设备中扮演着越来越重要的角色。
本文将对功率集成器件进行详细介绍,包括定义、分类、发展历程、关键技术、应用领域以及未来发展趋势和挑战。
【功率集成器件的定义和分类】功率集成器件是一种将多种电力电子器件功能集成于一体的器件,主要包括功率晶体管、功率二极管、晶闸管、IGBT等。
根据不同的功能和应用,功率集成器件可分为电压控制型、电流控制型和混合型等。
【功率集成器件的发展历程】功率集成器件的发展经历了几个阶段,从20世纪50年代的真空管,到60年代的硅控整流器(SCR)、晶闸管,再到70年代的功率晶体管、功率二极管等。
近年来,随着制造工艺的进步,IGBT等新型功率集成器件逐渐成为主流。
【功率集成器件的关键技术和应用领域】功率集成器件的关键技术包括器件结构设计、制造工艺、封装技术等。
在应用领域方面,功率集成器件广泛应用于工业控制、家用电器、交通运输、新能源等领域。
【我国在功率集成器件领域的发展现状】我国在功率集成器件领域取得了一定的成绩,尤其在晶闸管、功率晶体管等领域具有较高的市场份额。
然而,与国外先进水平相比,我国在新型功率集成器件如IGBT等方面仍存在一定差距。
【功率集成器件的未来发展趋势和挑战】未来,功率集成器件将向更高电压、更高电流、更低损耗、更小型化等方向发展。
同时,新型材料、封装技术和制造工艺的不断突破将为功率集成器件带来更多可能。
在挑战方面,提高器件性能、降低制造成本、增强可靠性等仍然是亟待解决的问题。
高速MOSFET栅极驱动电路的设计与应用指南
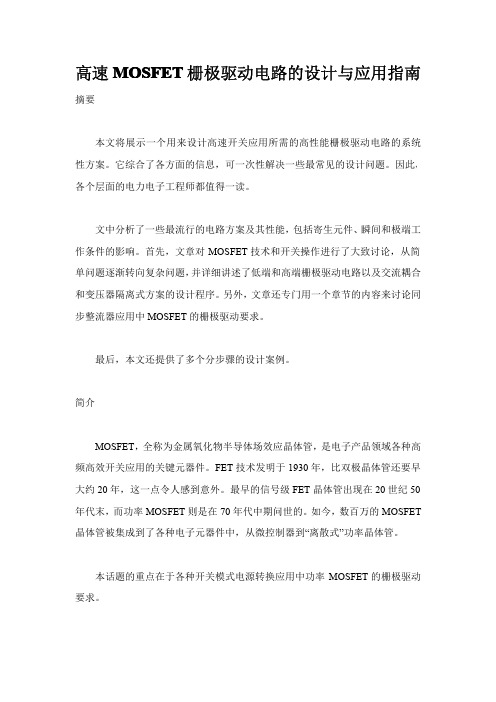
高速MOSFEMOSFET T栅极驱动电路的设计与应用指南摘要本文将展示一个用来设计高速开关应用所需的高性能栅极驱动电路的系统性方案。
它综合了各方面的信息,可一次性解决一些最常见的设计问题。
因此,各个层面的电力电子工程师都值得一读。
文中分析了一些最流行的电路方案及其性能,包括寄生元件、瞬间和极端工作条件的影响。
首先,文章对MOSFET技术和开关操作进行了大致讨论,从简单问题逐渐转向复杂问题,并详细讲述了低端和高端栅极驱动电路以及交流耦合和变压器隔离式方案的设计程序。
另外,文章还专门用一个章节的内容来讨论同步整流器应用中MOSFET的栅极驱动要求。
最后,本文还提供了多个分步骤的设计案例。
简介MOSFET,全称为金属氧化物半导体场效应晶体管,是电子产品领域各种高频高效开关应用的关键元器件。
FET技术发明于1930年,比双极晶体管还要早大约20年,这一点令人感到意外。
最早的信号级FET晶体管出现在20世纪50年代末,而功率MOSFET则是在70年代中期问世的。
如今,数百万的MOSFET 晶体管被集成到了各种电子元器件中,从微控制器到“离散式”功率晶体管。
本话题的重点在于各种开关模式电源转换应用中功率MOSFET的栅极驱动要求。
Design And Application GuideFor High Speed MOSFET Gate Drive CircuitsBy Laszlo BaloghABSTRACTThe main purpose of this paper is to demonstrate a systematic approach to design high performance gate drive circuits for high speed switching applications. It is an informative collection of topics offering a “one-stop-shopping” to solve the most common design challenges. Thus it should be of interest to power electronics engineers at all levels of experience.The most popular circuit solutions and their performance are analyzed, including the effect of parasitic components, transient and extreme operating conditions. The discussion builds from simple to more complex problems starting with an overview of MOSFET technology and switching operation. Design procedure for ground referenced and high side gate drive circuits, AC coupled and transformer isolated solutions are described in great details. A special chapter deals with the gate drive requirements of the MOSFETs in synchronous rectifier applications.Several, step-by-step numerical design examples complement the paper.INTRODUCTIONMOSFET – is an acronym for Metal Oxide Semiconductor Field Effect Transistor and it is the key component in high frequency, high efficiency switching applications across the electronics industry. It might be surprising, but FET technology was invented in 1930, some 20 years before the bipolar transistor. The first signal level FET transistors were built in the late 1950’s while power MOSFETs have been available from the mid 70’s. Today, millions of MOSFET transistors are integrated in modern electronic components, from microprocessors, through “discrete” power transistors.The focus of this topic is the gate drive requirements of the power MOSFET in various switch mode power conversion applications. MOSFET TECHNOLOGYThe bipolar and the MOSFET transistors exploit the same operating principle. Fundamentally, both type of transistors are charge controlled devices which means that their output current is proportional to the charge established in the semiconductor by the control electrode. When these devices are used as switches, both must be driven from a low impedance source capable of sourcing and sinking sufficient current to provide for fast insertion and extraction of the controlling charge. From this point of view, the MOSFETs have to be driven just as “hard” during turn-on and turn-off as a bipolar transistor to achieve comparable switching speeds. Theoretically, the switching speeds of the bipolar and MOSFET devices are close to identical, determined by the time required for the charge carriers to travel across the semiconductor region. Typical values in power devices are approximately 20 to 200 picoseconds depending on the size of the device. The popularity and proliferation of MOSFET technology for digital and power applications is driven by two of their major advantages over the bipolar junction transistors. One of these benefits is the ease of use of the MOSFET devices in high frequency switching applications. The MOSFET transistors are simpler to drive because their control electrode is isolated from the current conducting silicon, therefore a continuous ON current is not required. Once the MOSFET transistors are turned-on, their drive current is practically zero. Also, the controlling charge and accordingly the storage time in the MOSFET transistors is greatly reduced. This basically1eliminates the design trade-off between on state voltage drop – which is inversely proportional to excess control charge – and turn-off time. As a result, MOSFET technology promises to use much simpler and more efficient drive circuits with significant economic benefits compared to bipolar devices.Furthermore, it is important to highlight especially for power applications, that MOSFETs have a resistive nature. The voltage drop across the drain source terminals of a MOSFET is a linear function of the current flowing in the semiconductor. This linear relationship is characterized by the R DS(on) of the MOSFET and known as the on-resistance. On-resistance is constant for a given gate-to-source voltage and temperature of the device. As opposed to the -2.2mV/°C temperature coefficient of a p-n junction, the MOSFETs exhibit a positive temperature coefficient of approximately 0.7%/°C to 1%/°C. This positive temperature coefficient of the MOSFET makes it an ideal candidate for parallel operation in higher power applications where using a single device would not be practical or possible. Due to the positive TC of the channel resistance, parallel connected MOSFETs tend to share the current evenly among themselves. This current sharing works automatically in MOSFETs since the positive TC acts as a slow negative feedback system. The device carrying a higher current will heat up more – don’t forget that the drain to source voltages are equal – and the higher temperature will increase its R DS(on) value. The increasing resistance will cause the current to decrease, therefore the temperature to drop. Eventually, an equilibrium is reached where the parallel connected devices carry similar current levels. Initial tolerance in R DS(on) values and different junction to ambient thermal resistances can cause significant – up to 30% – error in current distribution.Device typesAlmost all manufacturers have got their unique twist on how to manufacture the best power MOSFETs, but all of these devices on the market can be categorized into three basic device types. These are illustrated in Figure 1.Figure 1. Power MOSFET device types Double-diffused MOS transistors were introduced in the 1970’s for power applications and evolved continuously during the years. Using polycrystalline silicon gate structures and self-aligning processes, higher density integration and rapid reduction in capacitances became possible. The next significant advancement was offered by the V-groove or trench technology to further increase cell density in power MOSFET devices. The better performance and denser integration don’t come free however, as trench MOS devices are more difficult to manufacture.The third device type to be mentioned here is the lateral power MOSFETs. This device type is constrained in voltage and current rating due to its inefficient utilization of the chip geometry. Nevertheless, they can provide significant benefits in low voltage applications, like in microprocessor power supplies or as synchronous rectifiers in isolated converters.2The lateral power MOSFETs have significantly lower capacitances, therefore they can switch much faster and they require much less gate drive power.MOSFET ModelsThere are numerous models available to illustrate how the MOSFET works, nevertheless finding the right representation might be difficult. Mostof the MOSFET manufacturers provide Spice and/or Saber models for their devices, but these models say very little about the application traps designers have to face in practice. They provide even fewer clues how to solve the most common design challenges.A really useful MOSFET model which would describe all important properties of the device from an application point of view would be very complicated. On the other hand, very simple and meaningful models can be derived of the MOSFET transistor if we limit the applicabilityof the model to certain problem areas.The first model in Figure 2 is based on the actual structure of the MOSFET device and can be used mainly for DC analysis. The MOSFET symbol in Figure 2a represents the channel resistance and the JFET corresponds to the resistance of the epitaxial layer. The length, thus the resistance of the epi layer is a function of the voltage rating of the device as high voltage MOSFETs require thicker epitaxial layer.Figure 2b can be used very effectively to model the dv/dt induced breakdown characteristic of a MOSFET. It shows both main breakdown mechanisms, namely the dv/dt induced turn-on of the parasitic bipolar transistor - present in all power MOSFETs - and the dv/dt induced turn-onof the channel as a function of the gate terminating impedance. Modern power MOSFETs are practically immune to dv/dt triggering of the parasitic npn transistor due to manufacturing improvements to reduce the resistance between the base and emitter regions.It must be mentioned also that the parasitic bipolar transistor plays another important role. Its base – collector junction is the famous body diode of the MOSFET.Figure 2. Power MOSFET models34Figure 2c is the switching model of the MOSFET. The most important parasitic components influencing switching performance are shown in this model. Their respective roles will be discussed in the next chapter which is dedicated to the switching procedure of the device.MOSFET Critical ParametersWhen switch mode operation of the MOSFET is considered, the goal is to switch between the lowest and highest resistance states of the device in the shortest possible time. Since the practical switching times of the MOSFETs (~10ns to 60ns) is at least two to three orders of magnitude longer than the theoretical switching time (~50ps to 200ps), it seems important to understand the discrepancy. Referring back to the MOSFET models in Figure 2, note that all models include three capacitors connected between the three terminals of the device. Ultimately, the switching performance of the MOSFET transistor is determined by how quickly the voltages can be changed across these capacitors.Therefore, in high speed switching applications, the most important parameters are the parasitic capacitances of the device. Two of these capacitors, the C GS and C GD capacitors correspond to the actual geometry of the device while the C DS capacitor is the capacitance of the base collector diode of the parasitic bipolar transistor (body diode).The C GS capacitor is formed by the overlap of the source and channel region by the gate electrode. Its value is defined by the actual geometry of the regions and stays constant (linear) under different operating conditions.The C GD capacitor is the result of two effects. Part of it is the overlap of the JFET region and the gate electrode in addition to the capacitance of the depletion region which is non-linear. The equivalent C GD capacitance is a function of the drain source voltage of the device approximated by the following formula:DS1GD,0GD V K 1C C ⋅+≈The C DS capacitor is also non-linear since it is the junction capacitance of the body diode. Its voltage dependence can be described as:DS 2DS,0DS V K C C ⋅≈Unfortunately, non of the above mentioned capacitance values are defined directly in the transistor data sheets. Their values are given indirectly by the C ISS , C RSS , and C OSS capacitor values and must be calculated as: RSSOSS DS RSS ISS GS RSSGD C C C C C C C C −=−== Further complication is caused by the C GD capacitor in switching applications because it is placed in the feedback path between the input and output of the device. Accordingly, its effective value in switching applications can be much larger depending on the drain source voltage of the MOSFET. This phenomenon is called the “Miller” effect and it can be expressed as:()GD L fs eqv GD,C R g 1C ⋅⋅+=Since the C GD and C DS capacitors are voltage dependent, the data sheet numbers are valid only at the test conditions listed. The relevant average capacitances for a certain application have to be calculated based on the required charge to establish the actual voltage change across the capacitors. For most power MOSFETs the following approximations can be useful: offDS,spec DS,spec OSS,ave OSS,off DS,spec DS,spec RSS,ave GD,V V C 2C V V C 2C ⋅⋅=⋅⋅=The next important parameter to mention is the gate mesh resistance, R G,I . This parasitic resistance describes the resistance associated by the gate signal distribution within the device. Its importance is very significant in high speed switching applications because it is in between the driver and the input capacitor of the device, directly impeding the switching times and the5dv/dt immunity of the MOSFET. This effect is recognized in the industry, where real high speed devices like RF MOSFET transistors use metal gate electrodes instead of the higher resistance polysilicon gate mesh for gate signal distribution. The R G,I resistance is not specified in the data sheets, but in certain applications it can be a very important characteristic of the device. In the back of this paper, Appendix A4 shows a typical measurement setup to determine the internal gate resistor value with an impedance bridge.Obviously, the gate threshold voltage is also a critical characteristic. It is important to note that the data sheet V TH value is defined at 25°C and at a very low current, typically at 250μA. Therefore, it is not equal to the Miller plateau region of the commonly known gate switching waveform. Another rarely mentioned fact about V TH is its approximately –7mV/°C temperature coefficient. It has particular significance in gate drive circuits designed for logic level MOSFET where V TH is already low under the usual test conditions. Since MOSFETs usually operate at elevated temperatures, proper gate drive design must account for the lower V TH when turn-off time, and dv/dt immunity is calculated as shown in Appendix A and F.The transconductance of the MOSFET is its small signal gain in the linear region of its operation. It is important to point out that every time the MOSFET is turned-on or turned-off, it must go through its linear operating mode where the current is determined by the gate-to-source voltage. The transconductance, g fs , is the small signal relationship between drain current and gate-to-source voltage:GSD fs dV dI g =Accordingly, the maximum current of the MOSFET in the linear region is given by: ()fs th GS D g V V I ⋅−=Rearranging this equation for V GS yields the approximate value of the Miller plateau as a function of the drain current.fs D th Miller GS,g IV V +=Other important parameters like the source inductance (L S ) and drain inductance (L D ) exhibit significant restrictions in switching performance. Typical L S and L D values are listed in the data sheets, and they are mainly dependant on the package type of the transistor. Their effects can be investigated together with the external parasitic components usually associated with layout and with accompanying external circuit elements like leakage inductance, a current sense resistor, etc.For completeness, the external series gate resistor and the MOSFET driver’s output impedance must be mentioned as determining factors in high performance gate drive designs as they have a profound effect on switching speeds and consequently on switching losses.SWITCHING APPLICATIONSNow, that all the players are identified, let’s investigate the actual switching behavior of the MOSFET transistors. To gain a better understanding of the fundamental procedure, the parasitic inductances of the circuit will be neglected. Later their respective effects on the basic operation will be analyzed individually. Furthermore, the following descriptions relate to clamped inductive switching because most MOSFET transistors and high speed gate drive circuits used in switch mode power supplies work in that operating mode.Figure 3. Simplified clamped inductive switchingmodelThe simplest model of clamped inductive switching is shown in Figure 3, where the DC current source represents the inductor. Its current can be considered constant during the short switching interval. The diode provides a path for the current during the off time of the MOSFET and clamps the drain terminal of the device to the output voltage symbolized by the battery.Turn-On procedureThe turn-on event of the MOSFET transistor can be divided into four intervals as depicted in Figure 4.Figure 4. MOSFET turn-on time intervalsIn the first step the input capacitance of the device is charged from 0V to V TH. During this interval most of the gate current is charging the C GS capacitor. A small current is flowing through the C GD capacitor too. As the voltage increases at the gate terminal and the C GD capacitor’s voltage has to be slightly reduced. This period is called the turn-on delay, because both the drain current and the drain voltage of the device remain unchanged.Once the gate is charged to the threshold level, the MOSFET is ready to carry current. In the second interval the gate is rising from V TH to the Miller plateau level, V GS,Miller. This is the linear operation of the device when current is proportional to the gate voltage. On the gate side, current is flowing into the C GS and C GD capacitors just like in the first time interval and the V GS voltage is increasing. On the output side of the device, the drain current is increasing, while the drain-to-source voltage stays at the previous level (V DS,OFF). This can be understood looking at the schematic in Figure 3. Until all the current is transferred into the MOSFET and the diode is turned-off completely to be able to block reverse voltage across its pn junction, the drain voltage must stay at the output voltage level. Entering into the third period of the turn-on procedure the gate is already charged to the sufficient voltage (V GS,Miller) to carry the entire load current and the rectifier diode is turned off. That now allows the drain voltage to fall. While the drain voltage falls across the device, the gate-to-source voltage stays steady. This is the Miller plateau region in the gate voltage waveform. All the gate current available from the driver is diverted to discharge the C GD capacitor to facilitate the rapid voltage change across the drain-to-source terminals. The drain current of the device stays constant since it is now limited by the external circuitry, i.e. the DC current source.The last step of the turn-on is to fully enhance the conducting channel of the MOSFET by applying a higher gate drive voltage. The final amplitude of V GS determines the ultimate on-resistance of the device during its on-time. Therefore, in this fourth interval, V GS is increased from V GS,Miller to its final value, V DRV. This is accomplished by charging the C GS and C GD capacitors, thus gate current is now split between the two components. While these capacitors are being charged, the drain current is still constant, and the drain-to-source voltage is slightly decreasing as the on-resistance of the device is being reduced.6Turn-Off procedureThe description of the turn-off procedure for the MOSFET transistor is basically back tracking the turn-on steps from the previous section. Start with V GS being equal to V DRV and the current in the device is the full load current represented by I DC in Figure 3. The drain-to-source voltage is being defined by I DC and the R DS(on) of the MOSFET. The four turn-off steps are shown in Figure 5. for completeness.Figure 5. MOSFET turn-off time intervals The first time interval is the turn-off delay which is required to discharge the C ISS capacitance from its initial value to the Miller plateau level. During this time the gate current is supplied by the C ISS capacitor itself and it is flowing through the C GS and C GD capacitors of the MOSFET. The drain voltage of the device is slightly increasing as the overdrive voltage is diminishing. The current in the drain is unchanged.In the second period, the drain-to-source voltage of the MOSFET rises from I D⋅R DS(on) to the final V DS(off) level, where it is clamped to the output voltage by the rectifier diode according to the simplified schematic of Figure 3. During this time period – which corresponds to the Miller plateau in the gate voltage waveform - the gate current is strictly the charging current of the C GDcapacitor because the gate-to-source voltage is constant. This current is provided by the bypass capacitor of the power stage and it is subtracted from the drain current. The total drain current still equals the load current, i.e. the inductor current represented by the DC current source in Figure 3.The beginning of the third time interval is signified by the turn-on of the diode, thus providing an alternative route to the load current.The gate voltage resumes falling from V GS,Miller to V TH. The majority of the gate current is coming out of the C GS capacitor, because the C GDcapacitor is virtually fully charged from the previous time interval. The MOSFET is in linear operation and the declining gate-to-source voltage causes the drain current to decrease and reach near zero by the end of this interval.Meanwhile the drain voltage is steady at V DS(off)due to the forward biased rectifier diode.The last step of the turn-off procedure is to fully discharge the input capacitors of the device. V GSis further reduced until it reaches 0V. The bigger portion of the gate current, similarly to the third turn-off time interval, supplied by the C GScapacitor. The drain current and the drain voltage in the device are unchanged.Summarizing the results, it can be concluded that the MOSFET transistor can be switched between its highest and lowest impedance states (either turn-on or turn-off) in four time intervals. The lengths of all four time intervals are a function of the parasitic capacitance values, the required voltage change across them and the available gate drive current. This emphasizes the importance of the proper component selection and optimum gate drive design for high speed, high frequency switching applications.7Characteristic numbers for turn-on, turn-off delays, rise and fall times of the MOSFET switching waveforms are listed in the transistor data sheets. Unfortunately, these numbers correspond to the specific test conditions and to resistive load, making the comparison of different manufacturers’ products difficult. Also, switching performance in practical applications with clamped inductive load is significantly different from the numbers given in the data sheets.Power lossesThe switching action in the MOSFET transistorin power applications will result in some unavoidable losses, which can be divided into two categories.The simpler of the two loss mechanisms is the gate drive loss of the device. As described before, turning-on or off the MOSFET involves chargingor discharging the C ISS capacitor. When the voltage across a capacitor is changing, a certain amount of charge has to be transferred. The amount of charge required to change the gate voltage between 0V and the actual gate drive voltage V DRV, is characterized by the typical gate charge vs. gate-to-source voltage curve in the MOSFET datasheet. An example is shown in Figure 6.Figure 6. Typical gate charge vs. gate-to-sourcevoltage This graph gives a relatively accurate worst case estimate of the gate charge as a function of the gate drive voltage. The parameter used to generate the individual curves is the drain-to-source off state voltage of the device. V DS(off) influences the Miller charge – the area below the flat portion of the curves – thus also, the total gate charge required in a switching cycle. Once the total gate charge is obtained from Figure 6, the gate charge losses can be calculated as:DRVGDRVGATEfQVP⋅⋅=where V DRV is the amplitude of the gate drive waveform and f DRV is the gate drive frequency – which is in most cases equal to the switching frequency. It is interesting to notice that the Q G⋅f DRV term in the previous equation gives the average bias current required to drive the gate. The power lost to drive the gate of the MOSFET transistor is dissipated in the gate drive circuitry. Referring back to Figures 4 and 5, the dissipating components can be identified as the combination of the series ohmic impedances in the gate drive path. In every switching cycle the required gate charge has to pass through the driver output impedances, the external gate resistor, and the internal gate mesh resistance. As it turns out, the power dissipation is independent of how quickly the charge is delivered through the resistors. Using the resistor designators from Figures 4 and 5, the driver power dissipation can be expressed as:OFFDRV,ONDRV,DRVIG,GATELODRVGDRVLOOFFDRV,IG,GATEHIDRVGDRVHIONDRV,PPPRRRfQVR21PRRRfQVR21P+=++⋅⋅⋅⋅=++⋅⋅⋅⋅=In the above equations, the gate drive circuit is represented by a resistive output impedance and this assumption is valid for MOS based gate drivers. When bipolar transistors are utilized in the gate drive circuit, the output impedance becomes non-linear and the equations do not yield the correct answers. It is safe to assume that with low value gate resistors (<5Ω) most gate drive losses are dissipated in the driver. If R GATE is sufficiently large to limit I G below the output89current capability of the bipolar driver, the majority of the gate drive power loss is then dissipated in R GATE .In addition to the gate drive power loss, the transistors accrue switching losses in the traditional sense due to high current and high voltage being present in the device simultaneously for a short period. In order to ensure the least amount of switching losses, the duration of this time interval must be minimized. Looking at the turn-on and turn-off procedures of the MOSFET, this condition is limited to intervals 2 and 3 of the switching transitions in both turn-on and turn-off operation. These time intervals correspond to the linear operation of the device when the gate voltage is between V TH and V GS,Miller , causing changes in the current of the device and to the Miller plateau region when the drain voltage goes through its switching transition.This is a very important realization to properly design high speed gate drive circuits. It highlights the fact that the most important characteristic of the gate driver is its source-sink current capability around the Miller plateau voltage level. Peak current capability, which is measured at full V DRV across the driver’s output impedance, has very little relevance to the actual switching performance of the MOSFET. What really determines the switching times of the device is the gate drive current capability when the gate-to-source voltage, i.e. the output of the driver is at ~5V (~2.5V for logic level MOSFETs).A crude estimate of the MOSFET switching losses can be calculated using simplified linear approximations of the gate drive current, drain current and drain voltage waveforms during periods 2 and 3 of the switching transitions. First the gate drive currents must be determined for the second and third time intervals respectively:()G.I GATE HI MillerGS,DRV G3G.IGATE HI TH Miller GS,DRVG2R R R V V I R R R V V 0.5V I ++−=+++⋅−=Assuming that I G2 charges the input capacitor of the device from V TH to V GS,Miller and I G3 is the discharge current of the C RSS capacitor while the drain voltage changes from V DS(off) to 0V, the approximate switching times are given as:G3offDS,RSS G2THMillerGS,ISS I V C t3I V V C t2⋅=−⋅=During t2 the drain voltage is V DS(off) and the current is ramping from 0A to the load current, I L while in t3 time interval the drain voltage is falling from V DS(off) to near 0V. Again, using linear approximations of the waveforms, the power loss components for the respective time intervals can be estimated:Loff DS,Loff DS,I 2V T t3P32I V T t2P2⋅⋅=⋅⋅=where T is the switching period. The total switching loss is the sum of the two loss components, which yields the following simplifed expression:Even though the switching transitions are well understood, calculating the exact switching losses is almost impossible. The reason is the effect of the parasitic inductive components which will significantly alter the current and voltage waveforms, as well as the switching times during the switching procedures. Taking into account the effect of the different source and drain inductances of a real circuit would result in second order differential equations to describe the actual waveforms of the circuit. Since the variables, including gate threshold voltage, MOSFET capacitor values, driver output impedances, etc. have a very wide tolerance, the above described linear approximation seems to be a reasonable enough compromise to estimate switching losses in the MOSFET.Effects of parasitic componentsThe most profound effect on switching performance is exhibited by the source inductance. There are two sources for parasitic source inductance in a typical circuit, the sourceTt3t22I V P L DS(off)SW +⋅⋅=。
化学技术在电子行业中的应用指南
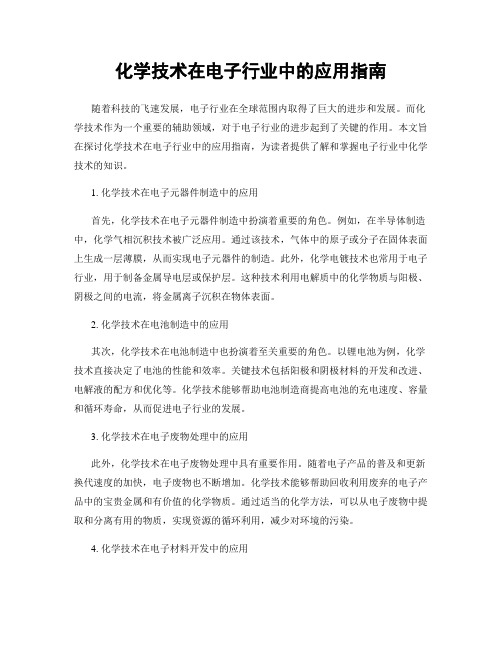
化学技术在电子行业中的应用指南随着科技的飞速发展,电子行业在全球范围内取得了巨大的进步和发展。
而化学技术作为一个重要的辅助领域,对于电子行业的进步起到了关键的作用。
本文旨在探讨化学技术在电子行业中的应用指南,为读者提供了解和掌握电子行业中化学技术的知识。
1. 化学技术在电子元器件制造中的应用首先,化学技术在电子元器件制造中扮演着重要的角色。
例如,在半导体制造中,化学气相沉积技术被广泛应用。
通过该技术,气体中的原子或分子在固体表面上生成一层薄膜,从而实现电子元器件的制造。
此外,化学电镀技术也常用于电子行业,用于制备金属导电层或保护层。
这种技术利用电解质中的化学物质与阳极、阴极之间的电流,将金属离子沉积在物体表面。
2. 化学技术在电池制造中的应用其次,化学技术在电池制造中也扮演着至关重要的角色。
以锂电池为例,化学技术直接决定了电池的性能和效率。
关键技术包括阳极和阴极材料的开发和改进、电解液的配方和优化等。
化学技术能够帮助电池制造商提高电池的充电速度、容量和循环寿命,从而促进电子行业的发展。
3. 化学技术在电子废物处理中的应用此外,化学技术在电子废物处理中具有重要作用。
随着电子产品的普及和更新换代速度的加快,电子废物也不断增加。
化学技术能够帮助回收利用废弃的电子产品中的宝贵金属和有价值的化学物质。
通过适当的化学方法,可以从电子废物中提取和分离有用的物质,实现资源的循环利用,减少对环境的污染。
4. 化学技术在电子材料开发中的应用最后,化学技术在电子材料开发中也具有重要作用。
电子材料的性能对于电子行业的发展至关重要。
化学技术可以帮助研究人员开发新型的电子材料,提高其导电性、稳定性和光学性能。
例如,通过合成具有特定结构和组分的纳米材料,可以制备出具有优异性能的电子材料,从而推动电子行业的不断创新和发展。
综上所述,化学技术在电子行业中起到了至关重要的作用。
从电子元器件制造到电池制造,从电子废物处理到电子材料开发,化学技术可以帮助提高产品的性能和质量,推动电子行业的发展和进步。
高速MOS驱动电路设计和应用指南

高速MOS驱动电路设计和应用指南摘要本篇论文的主要目的是来论证一种为高速开关应用而设计高性能栅极驱动电路的系统研究方法。
它是对“一站买齐”主题信息的收集,用来解决设计中最常见的挑战。
因此,各级的电力电子工程师对它都应该感兴趣。
对最流行电路解决方案和他们的性能进行了分析,这包括寄生部分的影响、瞬态的和极限的工作情况。
整篇文章开始于对MOSFET技术和开关工作的概述,随后进行简单的讨论然后再到复杂问题的分析。
仔细描述了设计过程中关于接地和高边栅极驱动电路、AC耦合和变压器隔离的解决方案。
其中一个章节专门来解决同步整流器应用中栅极驱动对MOSFET的要求。
另外,文章中还有一些一步一步的参数分析设计实例。
简介MOSFET是Metal Oxide Semiconductor Field Effect Transistor的首字母缩写,它在电子工业高频、高效率开关应用中是一种重要的元件。
或许人们会感到不可思议,但是FET是在1930年,大约比双极晶体管早20年被发明出来。
第一个信号电平FET晶体管制成于二十世纪60年代末期,而功率MOSFET是在二十世纪80年代开始被运用的。
如今,成千上万的MOSFET晶体管集成在现代电子元件,从微型的到“离散”功率晶体管。
本课题的研究重点是在各种开关模型功率转换应用中栅极驱动对功率MOSFET 的要求。
场效应晶体管技术双极晶体管和场效应晶体管有着相同的工作原理。
从根本上说,,两种类型晶体管均是电荷控制元件,即它们的输出电流和控制极半导体内的电荷量成比例。
当这些器件被用作开关时,两者必须和低阻抗源极的拉电流和灌电流分开,用以为控制极电荷提供快速的注入和释放。
从这点看,MOS-FET在不断的开关,当速度可以和双极晶体管相比拟时,它被驱动的将十分的‘激烈’。
理论上讲,双极晶体管和MOSFET的开关速度是基本相同的,这取决与载流子穿过半导体所需的时间。
在功率器件的典型值为20 ~ 200皮秒,但这个时间和器件的尺寸大小有关。
功率器件应用指南
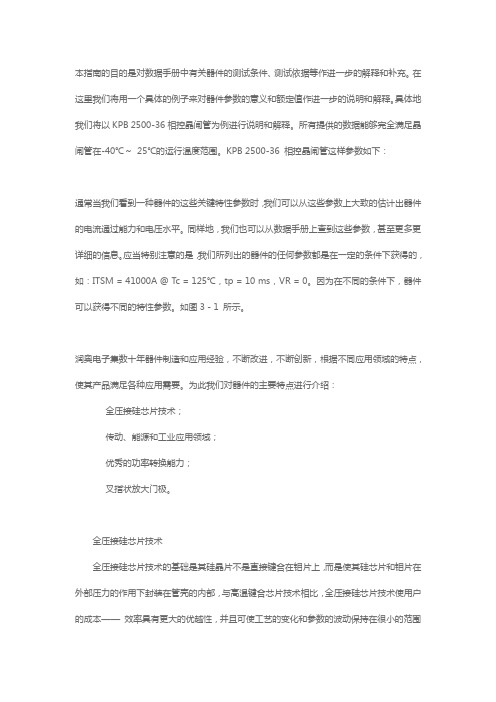
本指南的目的是对数据手册中有关器件的测试条件、测试依据等作进一步的解释和补充。
在这里我们将用一个具体的例子来对器件参数的意义和额定值作进一步的说明和解释。
具体地我们将以KPB 2500-36相控晶闸管为例进行说明和解释。
所有提供的数据能够完全满足晶闸管在-40℃~25℃的运行温度范围。
KPB 2500-36 相控晶闸管这样参数如下:通常当我们看到一种器件的这些关键特性参数时,我们可以从这些参数上大致的估计出器件的电流通过能力和电压水平。
同样地,我们也可以从数据手册上查到这些参数,甚至更多更详细的信息。
应当特别注意的是,我们所列出的器件的任何参数都是在一定的条件下获得的,如:ITSM = 41000A @ Tc = 125℃,tp = 10 ms,VR = 0。
因为在不同的条件下,器件可以获得不同的特性参数。
如图3-1 所示。
润奥电子集数十年器件制造和应用经验,不断改进,不断创新,根据不同应用领域的特点,使其产品满足各种应用需要。
为此我们对器件的主要特点进行介绍:全压接硅芯片技术;传动、能源和工业应用领域;优秀的功率转换能力;叉指状放大门极。
全压接硅芯片技术全压接硅芯片技术的基础是其硅晶片不是直接键合在钼片上,而是使其硅芯片和钼片在外部压力的作用下封装在管壳的内部,与高温键合芯片技术相比,全压接硅芯片技术使用户的成本——效率具有更大的优越性,并且可使工艺的变化和参数的波动保持在很小的范围内,这样的话可使得器件的静态和动态特性得到优化。
传动、能源和工业应用领域因为上面的每一种应用均有其对半导体器件的特殊要求,TEG数十年内已提供了许多的器件到这些领域应用,这使得TEG 在满足客户的需求方面获得了独特的经验,其失效率特别低。
优化功能转换能力上面已经谈到,TEG获得了许多满足用户需要的独特经验,因此在我们的设计中特别注意作为工程应用时在静态和动态参数之间取得满意的优化组合,尤其像工作在较高频率的特殊应用情况时。
电气工程中的电力电子器件规范要求与选型指南
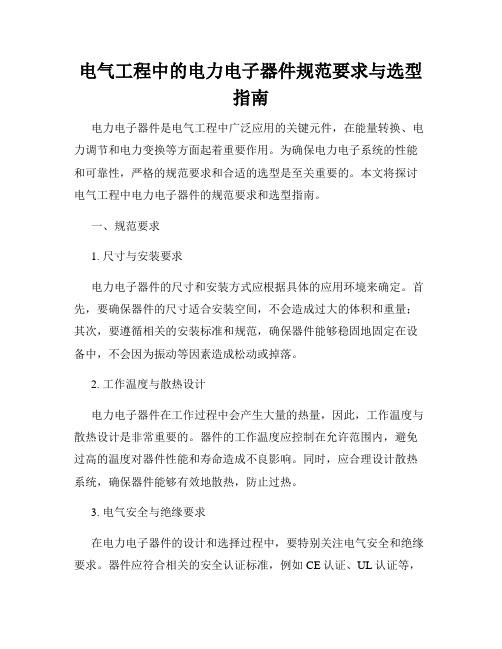
电气工程中的电力电子器件规范要求与选型指南电力电子器件是电气工程中广泛应用的关键元件,在能量转换、电力调节和电力变换等方面起着重要作用。
为确保电力电子系统的性能和可靠性,严格的规范要求和合适的选型是至关重要的。
本文将探讨电气工程中电力电子器件的规范要求和选型指南。
一、规范要求1. 尺寸与安装要求电力电子器件的尺寸和安装方式应根据具体的应用环境来确定。
首先,要确保器件的尺寸适合安装空间,不会造成过大的体积和重量;其次,要遵循相关的安装标准和规范,确保器件能够稳固地固定在设备中,不会因为振动等因素造成松动或掉落。
2. 工作温度与散热设计电力电子器件在工作过程中会产生大量的热量,因此,工作温度与散热设计是非常重要的。
器件的工作温度应控制在允许范围内,避免过高的温度对器件性能和寿命造成不良影响。
同时,应合理设计散热系统,确保器件能够有效地散热,防止过热。
3. 电气安全与绝缘要求在电力电子器件的设计和选择过程中,要特别关注电气安全和绝缘要求。
器件应符合相关的安全认证标准,例如CE认证、UL认证等,以保证产品的安全性和可靠性。
此外,还应注意器件的绝缘性能,确保在高电压环境下不会发生漏电或击穿等事故。
4. 电流、电压与功率要求电力电子器件的选型应根据实际的电流、电压和功率要求来确定。
对于大功率电子器件,要选择能够承受相应电流和电压的器件,并且要根据工作条件计算和评估器件的功率损耗和效率,确保其能够满足系统的要求。
二、选型指南1. 具体应用需求在选择电力电子器件时,首先要明确具体的应用需求,包括输入和输出电压、电流、功率要求,以及工作温度范围等。
只有明确了这些要求,才能有针对性地选择合适的器件。
2. 稳定性与可靠性电力电子器件的稳定性和可靠性是非常重要的因素。
选型时要考虑器件的质量和可靠性指标,例如寿命、可靠性参数等。
同时,还要了解器件的热稳定性和温度系数,以确保在不同的温度下其性能稳定。
3. 效率与功率损耗电力电子器件的效率和功率损耗直接影响整个系统的能效和经济性。
几种常用的功率器件(电力半导体)及其应用
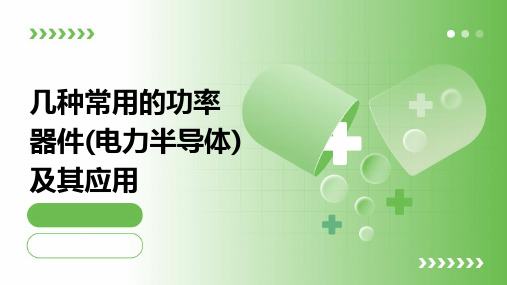
目录
• 引言 • 几种常用功率器件介绍 • 电力半导体器件工作原理及特性 • 几种常用功率器件应用领域探讨 • 选型指南与使用注意事项 • 总结与展望
01
引言
背景与意义
功率器件是电力电子 技术的核心,广泛应 用于能源、交通、工 业等领域
功率器件的性能和可 靠性对电力电子系统 的效率和稳定性具有 重要影响
随着新能源、电动汽 车等产业的快速发展, 功率器件的需求不断 增长
功率器件概述
1
功率器件是一种能够控制、转换和传输电能的半 导体器件
2
主要类型包括二极管、晶体管、晶闸管、 MOSFET、IGBT等
3
功率器件具有耐压高、耐流大、开关速度快等特 点,是实现电力电子变换的关键元件
02
几种常用功率器件介绍
注意器件的开关顺序和时序
不正确的开关顺序或时序可能会导致电路故障或器件损坏。
确保良好的散热条件
功率器件在工作时会产生热量,需要确保良好的散热条件以防止器件 过热损坏。
06
总结与展望
回顾本次项目成果
深入研究了几种常用的功率器件(电力半导体)的工作原理和特性,包括晶 闸管、可关断晶闸管、电力晶体管、绝缘栅双极晶体管等。
描述器件在异常工作条件下的承受能力, 如过压、过流、过热等保护功能,确保器 件在恶劣环境下能够安全运行。
04
几种常用功率器件应用领 域探讨
电源供应器与适配器
开关电源
功率器件如MOSFET和IGBT在开 关电源中起到关键作用,实现高 效能、小体积的电源设计。
适配器
功率器件用于电压转换和电流控 制,使得适配器能够为各种设备 提供稳定的电源。
晶闸管应用指南
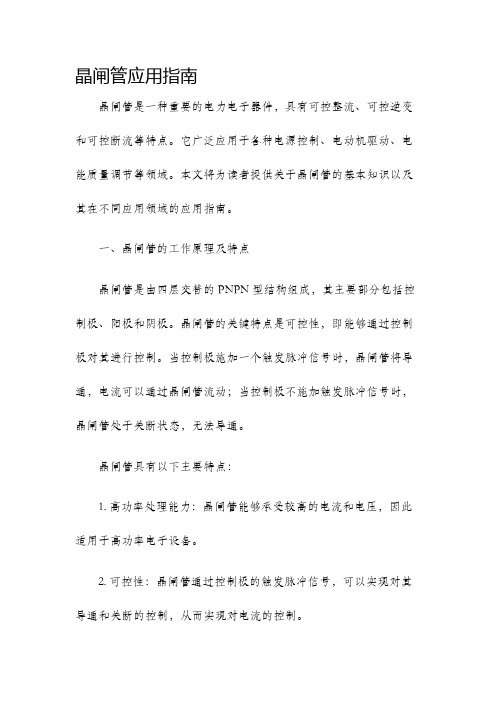
晶闸管应用指南晶闸管是一种重要的电力电子器件,具有可控整流、可控逆变和可控断流等特点。
它广泛应用于各种电源控制、电动机驱动、电能质量调节等领域。
本文将为读者提供关于晶闸管的基本知识以及其在不同应用领域的应用指南。
一、晶闸管的工作原理及特点晶闸管是由四层交替的PNPN型结构组成,其主要部分包括控制极、阳极和阴极。
晶闸管的关键特点是可控性,即能够通过控制极对其进行控制。
当控制极施加一个触发脉冲信号时,晶闸管将导通,电流可以通过晶闸管流动;当控制极不施加触发脉冲信号时,晶闸管处于关断状态,无法导通。
晶闸管具有以下主要特点:1. 高功率处理能力:晶闸管能够承受较高的电流和电压,因此适用于高功率电子设备。
2. 可控性:晶闸管通过控制极的触发脉冲信号,可以实现对其导通和关断的控制,从而实现对电流的控制。
3. 快速开关速度:晶闸管开关速度快,能够在短时间内完成导通和关断操作。
4. 可靠性高:晶闸管结构简单,不易损坏,具有较高的可靠性。
二、晶闸管的应用领域晶闸管广泛应用于各种领域,以下介绍其中几个重要的应用领域:1. 电源控制:晶闸管可以用作交流电源控制器,实现对交流电源的整流、变压和稳压等控制,为各种电子设备提供可靠的电源。
2. 电机控制:晶闸管可以用作电机驱动器,实现对电机的启动、停止、调速和反向等控制,广泛应用于工业自动化和机械设备。
3. 电能质量调节:晶闸管可以用于电能质量调节,如电压调节、电流调节和功率因数校正等,提高电力系统的稳定性和可靠性。
4. 频率变换器:晶闸管可以用于频率变换器,将输入电源的频率转换成输出电源的频率,适用于交流电机调速和无级调速等应用。
5. 电力传输与分配:晶闸管可以用于电力传输和分配,实现对电力系统的电压和电流的控制和平衡。
- 1、下载文档前请自行甄别文档内容的完整性,平台不提供额外的编辑、内容补充、找答案等附加服务。
- 2、"仅部分预览"的文档,不可在线预览部分如存在完整性等问题,可反馈申请退款(可完整预览的文档不适用该条件!)。
- 3、如文档侵犯您的权益,请联系客服反馈,我们会尽快为您处理(人工客服工作时间:9:00-18:30)。
目录电力电子器件应用指南 (1)晶闸管、二极管主要参数及其含义 (8)晶闸管、二极管简易测试方法 (11)中频感应加热电源常见故障与维修 (13)水冷散热器的安装与使用 (20)晶闸管水冷散热器重复使用中应注意的问题 (23)电焊机用晶闸管模块的选择与应用 (25)电力半导体器件用散热器选择及使用原则 (32)风冷散热器的选配 (34)高频晶闸管新特性 (36)改进的晶闸管高di/dt性能 (39)门极触发强度对晶闸管开通特性的影响 (42)晶闸管串、并联配对选择及使用要求 (47)晶闸管在低温条件下的使用 (52)功率器件技术与电源技术的现状和发展 (53)晶闸管保护电路 (60)电力电子器件应用指南一、参数说明1本手册参数表中所给出的数据,I TSM、I2t、dv/dt、di/dt指的是元件所能满足的最小值,Q r、V TM、V TO、r T指元件可满足(不超过)的最大值。
2通态平均电流额定值I TAV(I FAV)I TAV(I FAV)指在双面冷却条件下,在规定的散热器温度时,允许元件流过的最大正弦半波电流平均值。
I TAV(I FAV)对应元件额定有效值I RMS=1.57 I TAV。
实际使用中,若不能保证散热器温度低于规定值,或散热器与元件接触热阻远大于规定值,则元件应降额使用。
3晶闸管通态电流上升率di/dt参数表中所给的为元件通态电流上升率的临界重复值。
其对应不重复测试值为重复值的2倍以上,在使用过程中,必须保证元件导通期任何时候的电流上升率都不能超过其重复值。
4晶闸管使用频率晶闸管可工作的最大频率由其工作时的电流脉冲宽度t p,关断时间t q以及从关断后承受正压开始至其再次开通的时间t V决定。
f max=1/(t q+t p+t V)。
根据工作频率选取元件时必须保证元件从正向电流过零至开始承受正压的时间间隔t H>t q,并留有一定的裕量。
随着工作频率的升高,元件正向损耗E pf和反向恢复损耗E pr随之升高,元件通态电流须降额使用。
二、元件的选择正确地选择晶闸管、整流管等电力电子器件对保证整机设备的可靠性及降低设备成本具有重要意义。
元件的选择要综合考虑其使用环境、冷却方式、线路型式、负载性质等因素,在保证所选元件各参数具有裕量的条件下兼顾经济性。
由于电力电子器件的应用领域十分广泛,具体应用形式多种多样,下面仅就晶闸管元件在整流电路和单项中频逆变电路中的选择加以说明。
1.整流电路器件选择工频整流是晶闸管元件最常用的领域之一。
元件选用主要考虑其额定电压和额定电流。
(1)晶闸管器件的正反向峰值电压V DRM和V RRM:应为元件实际承受最大峰值电压U M的2-3倍,即V DRM/RRM=(2-3)U M。
各种整流线路对应的U M值见表1。
(2)晶闸管器件的额定通态电流I T(AV):晶闸管的I T(AV)值指的是工频正弦半波平均值,其对应的有效值I TRMS=1.57I T(AV)。
依照发热相等的原则,按实际流经器件的电流所产生的功耗小于器件允许最大功耗的原则选取器件,在简单计算中,可近似按实际电流有效值≤1.57I T(AV)来选择。
为使元件在工作过程中不因过热损坏,流经元件的实际有效值应在乘以安全系数1.5-2后才能等于1.57I T(AV)。
假设整流电路负载平均电流为I d,流经每个器件的电流有效值为KI d,则所选器件的额定通态电流应为:I T(AV)=(1.5-2)KI d/ 1.57=K fb I dK fb为计算系数。
对于控制角α=0O时,各种整流电路下的K fb值见表1。
选择元件I T值还应考虑元件散热方式。
一般情况下风冷比水冷相同元件的额定电流值要低;(AV)自然冷却情况下,元件的额定电流要降为标准冷却条件下的三分之一。
表1:整流器件的最大峰值电压U M及通态平均电流计算系数K fb注:U2为主回路变压器二次相电压有效值,单项半波电感负载电路带续流二极管。
2.中频逆变元件的选择一般400Hz以上的工作条件下,应考虑使用KK器件;频率在4KHz以上时,可考虑使用KA器件。
这里主要介绍一下并联逆变电路中元件的选择(见图一)。
(1)元件正向和反向峰值电压V DRM、V RRM元件正向和反向峰值电压应取其实际承受最大正、反向峰值电压的1.5-2倍。
假设逆变器直流输入电压为U d,功率因数为cosψ则:V DRM/RRM=(1.5-2)πU d /(2cosψ)(2)元件的额定通态电流I T(AV)考虑到元件在较高频率下工作时,其开关损耗非常显著,元件的额定通态电流应按实际流过其有效值I的2-3倍来考虑,即I T(AV)=(2-3)I/1.57假设逆变器直流输入电流为I d,则所选器件I T(AV)为I T(AV)=(2-3)×I d/(1.57 )=(0.9-1.5) ×I d(3)关断时间t q并联逆变线路中,KK元件的关断时间选择要根据触发引前时间t f和换流时间t r来决定。
一般取:t q=(t f-t r)×(当功率因数为0.8时t f约为周期的十分之一,t r按元件di/dt小于或等于100A/μS 来确定)在频率较高时,可通过减小换流时间t r,并适当牺牲功率因数增加t f的方法来选择具有合适t q值的元件以上简略介绍了整流和逆变工作条件下元件的选择。
在许多情况下,除了元件的额定电压、电流外,还要根据具体条件选择元件的门极参数、通态压降以及断态电压临界上升率dv/dt和通态电流临界上升率di/dt。
三、元件的保护晶闸管元件的电压和电流过载能力极差,尤其是耐压能力,瞬时的过压就会造成元件永久性的损坏。
为了使元件能长期可靠地运行,必须针对过压和过电流发生的原因采取保护措施。
1.过压保护晶闸管工作过程中可能承受的过压主要有以下几种:一种是由于装置拉、合闸、负载打火等引起的过压;一种是由于元件关断时产生的关断电压;还有因雷击等原因从电网侵入的浪涌电压。
为限制过电压的幅值低于元件的正反向峰值电压,可采取以下保护措施(见图二)。
(1)在变压器一次侧接上避雷器,在二次侧加装阻容保护、硒堆、压敏电阻等非线性电阻元件进行保护。
在整流直流侧采取压敏电阻和泄能保护装置,以防止元件承受过电压。
(2)在晶闸管阴阳极两端直接进行保护。
晶闸管关断过程中主电流过零反向后迅速由反向峰值恢复至零电流,此过程可在元件两端产生达正常工作峰值电压5-6倍的尖峰电压。
一般建议在尽可能靠近元件本身的地方接上阻容吸收回路。
电阻R选无感电阻,通常取5-30Ω;电容C通常在0.1-1μF,耐压选元件耐压的1.1-1.5倍。
具体R、C取值可根据元件型号及工作情况调试决定。
注意保证电阻R的功率,尤其在中频逆变电路中,使之不会因发热而损坏。
2.过流保护晶闸管元件在短时间内具有一定的过流能力,但在过流严重时,不采取保护措施,就会造成元件损坏。
在线路设计和元件选择时应考虑负载短路和过载情况,确保在异常情况下设备能自动保护。
一般有以下几种措施(见图三)。
(1)在进线中串接电抗器限制短路电流,使其他保护方式切断电流前元件短时间内不致损坏;(2)线路采用过流检测装置,由过流信号控制触发器抑制过流,或接入过流继电器。
(3)安装快速熔断器。
快速熔断器的动作时间要求在10ms以内,熔断体的额定电流I KR可按以下原则选取:1.57I T(AV)≥I KR≥I TI T(AV)为元件额定电流,I T为元件实际工作电流有效值四、晶闸管门极触发参数表中所给晶闸管I GT、V GT为能触发元件至通态的最小值,实际使用中,晶闸管门极触发I GT、V GT应远大于此值。
应用中门极触发电流波形对晶闸管开通时间、开通损耗以及di/dt承受能力,都有较大影响。
为保证元件工作在最佳状态,并增强抗干扰性能,对台基公司所有晶闸管,建议门极触发脉冲电流幅值:I GM=2~5A(<10A),上升率:di G/dt≥2A/µs,上升时间:t r≤1µs。
即采用极陡前沿的强触发脉冲(见图四)。
五、元件串并联使用元件串并联使用时,线路上应采取门极强触发脉冲、均流、均压措施,还须挑选开通、恢复特性一致的元件。
特别是元件串联工作于较高di/dt的逆变线路中时,其反向恢复特性对动态均压起主要作用。
六、散热器与元件的安装元件的冷却方式有加装散热器自然冷却,风冷和水冷等方式,为了使元件充分地发挥其额定性能并加强使用中的可靠性,除必须科学地选择散热器外还需正确地安装。
只有正确地安装散热器才能保证其与元件芯片间的热阻R j-hs满足数据表中的要求。
在元件与散热器的安装时,应注意以下事项:1.散热器的台面必须与元件台面尺寸相匹配,防止压扁、压歪损坏器件。
2.散热器台面必须具有较高的平整、光洁度。
建议散热器台面粗糙度小于或等于1.6μm,平整度小于或等于30μm。
安装时元件台面与散热器台面应保持清洁干净无油污等脏物。
3.安装时要保证元件台面与散热器的台面完全平行、同心。
安装过程中,要求通过元件中心线施加压力以使压力均匀分布在整个接触区域。
用户手工安装时,建议使用扭矩扳手,对所有紧固螺母交替均匀用力,压力的大小要达到数据表中的要求。
4.在重复使用水冷散热器时,应特别注意检查其台面是否光洁、平整,水腔内是否有水垢和堵塞,尤其注意台面是否出现下陷情况,若出现了上述情况应予以更换。
水冷散热器安装图见下图(图五):在使用中需注意,风冷方式加装散热器后,一般要求风速不低于6m/s;水冷方式要求水冷散热器水流量不小于4×103 ml/min,进水温度5℃-35℃,水质ρ≤2.5KΩc m。
台基公司可提供SS水冷系列和SF风冷系列以及各类非标及组件散热器,为元件配套使用。
根据元件通态额定平均电流推荐配置的标准型散热器型号见下表。
其中SF系列风冷散热器是指在强迫风冷(风速≥6m/s)条件下的推荐配置,用户在使用时应根据实际散热条件并考虑可靠性要求进行选择。
对于1000A以上元件一般不推荐使用风冷散热器,若使用风冷散热器,则元件额定电流需降额使用。
用户未作特别要求时,台基公司所提供的成套元件都按标准配置安装散热器。
晶闸管、二极管主要参数及其含义IEC标准中用来表征晶闸管、二极管性能、特点的参数有数十项,但用户经常用到的有十项左右,本文就晶闸管、二极管的主要参数做一简单介绍。
1.正向平均电流I F(AV)( 整流管)通态平均电流I T(AV)( 晶闸管)是指在规定的散热器温度T HS或管壳温度T C时,允许流过器件的最大正弦半波电流平均值。
此时,器件的结温已达到其最高允许温度T jm。
台基公司产品手册中均给出了相应通态电流对应的散热器温度T HS或管壳温度T C值,用户使用中应根据实际通态电流和散热条件来选择合适型号的器件。