MuCell technology for injection molding
MIM技术介绍
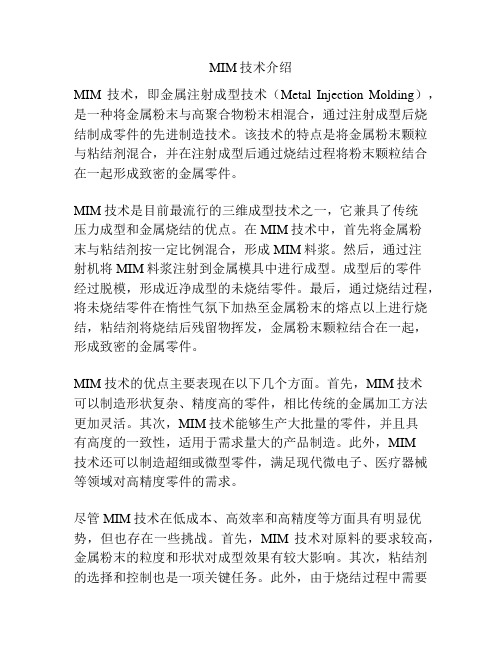
MIM技术介绍MIM技术,即金属注射成型技术(Metal Injection Molding),是一种将金属粉末与高聚合物粉末相混合,通过注射成型后烧结制成零件的先进制造技术。
该技术的特点是将金属粉末颗粒与粘结剂混合,并在注射成型后通过烧结过程将粉末颗粒结合在一起形成致密的金属零件。
MIM技术是目前最流行的三维成型技术之一,它兼具了传统压力成型和金属烧结的优点。
在MIM技术中,首先将金属粉末与粘结剂按一定比例混合,形成MIM料浆。
然后,通过注射机将MIM料浆注射到金属模具中进行成型。
成型后的零件经过脱模,形成近净成型的未烧结零件。
最后,通过烧结过程,将未烧结零件在惰性气氛下加热至金属粉末的熔点以上进行烧结,粘结剂将烧结后残留物挥发,金属粉末颗粒结合在一起,形成致密的金属零件。
MIM技术的优点主要表现在以下几个方面。
首先,MIM技术可以制造形状复杂、精度高的零件,相比传统的金属加工方法更加灵活。
其次,MIM技术能够生产大批量的零件,并且具有高度的一致性,适用于需求量大的产品制造。
此外,MIM技术还可以制造超细或微型零件,满足现代微电子、医疗器械等领域对高精度零件的需求。
尽管MIM技术在低成本、高效率和高精度等方面具有明显优势,但也存在一些挑战。
首先,MIM技术对原料的要求较高,金属粉末的粒度和形状对成型效果有较大影响。
其次,粘结剂的选择和控制也是一项关键任务。
此外,由于烧结过程中需要控制温度和气氛等因素,烧结工艺相对复杂。
因此,MIM技术的成功应用需要综合考虑材料、工艺和设备等多个因素。
总的来说,MIM技术是一种高度灵活、高效率、高精度的金属成型方法,已在汽车、航空航天、电子、医疗器械等领域得到广泛应用。
随着材料科学和制造技术的不断发展,MIM技术将进一步完善和推广,为各个行业提供更多高质量的金属零件。
MIM技术作为一种金属粉末成型技术,具有独特的优势和特点,逐渐成为制造业中不可忽视的一种先进工艺。
Mucell模具技术应用
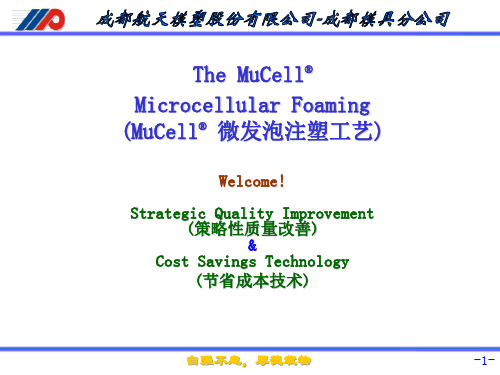
-13-
More Even Shrinkage in Part 更平均收缩率
自强不息,厚德载物
-14-
Improved Dimensional Stability 更高尺寸稳定性
自强不息,厚德载物
-15-
Improved Warpage 减少翘曲
自强不息,厚德载物
-16-
Better Part & Mold Design 更灵活的产品和模具设计
自强不息,厚德载物
-10-
Improved Flow Length 增加流动长度
自强不息,厚德载物
-11-
Reduced Cycle Time – no pack & hold 缩短注塑周期 –没有保压
自强不息,厚德载物
-12-
Lower Cavity Pressure 降低模穴压力
自强不息,厚德载物
自强不息,厚德载物
-3-
MuCell® Process Has Broad Machinery Builder Support (MuCell® 已广泛地被世界主要的注塑机品牌所支持)
A molder can retrofit an existing injection system for MuCell®. (注塑工厂可改造其现有的注塑机以使用MuCell®) 自强不息,厚德载物 -4-
1. 2. 3. Status (发展现状) Process (制程原理) Characteristics & Benefits (特点和贡献)
自强不息,厚德载物
-2-
MuCell® Development (MuCell®技术的发展)
1. Invented at Massachusetts Institute of Technology M.I.T. (早期 由麻省理工学院发明) 2. Commercial development completed by Trexel under worldwide exclusive license from M.I.T. (麻省理工学院独家全球授权美国 Trexel公司将技术商品化) 3. MuCell® is a proprietary technology available only from Trexel (MuCell® 技术仅由Trexel公司独家授权提供) 4. Over 70 patents filed in Asia, Europe and N. America(在亚洲,欧 洲及北美已拥有或申请超过70个专利权)
【机械专业中文翻译】模具设计对微孔泡沫注塑技术MuCell生产的聚合物孔结构的影响

模具设计对微孔泡沫注塑技术MuCell生产的聚合物孔结构的影响摘要在这项研究中,两副模具都用微孔泡沫注塑技术MuCell设计和使用以生成具有多孔结构的植入物。
为了到达所需孔隙结构,对许多工艺参数进行了调查用以说明工艺参数对孔形态的影响。
这个过程参数的调查分别在每个模具上进行严格地实验,这样模具设计对孔形态的影响可以通过相同工艺参数设置来研究。
于是研究发现,模具的设计对MuCell技术的孔隙结构确有影响。
一个适当的模具设计能提高生成的孔隙结构,比如孔隙率,孔径等,并且互相影响。
关键词: 模具设计,细胞形态,微孔泡沫注塑,注塑成型,医疗植入物,多孔聚合物,聚氨酯。
引言MuCell技术,作为一种有效的微孔注塑加工,被广泛应用于汽车和家具等行业。
采用微孔泡沫注塑技术在很多情况下可以节省原料,并且它可以用于生产具有多孔结构的封闭的产品[1]。
这项技术使用CO2作为发泡剂,并将其注入到注射机的塑化部分(图1)。
在供气线路和喷油器的作用下,被注入的发泡剂达到在其超临界状态在在注塑机的塑化部分成为熔体聚合物。
经过塑化的聚合物熔体和气体混合并通过喷嘴注入到模具内部,在模具内快速压降的影响下就变成了泡沫结构。
如今微孔泡沫注塑技术已经可以生产接近蜂窝形状的泡沫。
MuCell技术的微孔发泡注塑成型技术是一个完整的工艺和设备技术,有利于质量非常高,大大降低了生产成本。
MuCell工艺涉及在其超临界状态下气体的控制使用,以创建一个泡沫的一部分。
MuCell技术是有针对性的技术精度和工程塑料元件,最大墙壁厚度小于3mm。
MuCell工艺质量的关键措施,如平整度,圆度和翘曲,也消除了所有的凹痕,一般都提供了一个提高50-75%。
这些改进的结果,在成型零件,而不是固体成型的非均匀应力特性建立相对统一的应力模式。
作为被淘汰,因为群雄并持有成型周期阶段的MuCell工艺的均匀应力和收缩的直接结果(发生),所生产的零件往往更为密切的合作符合模具的形状,大概,部分本身的尺寸规格。
Mucell New Trend

) MuCell® lowers the viscosity of thermoplastic resins, hence offers longer flow length or material substitution
) MuCell®降低热塑性塑料的粘性,从而带来更长的流动长度。
Key MuCell® Results 使用MuCell® 后改善结果:
1. Typical weight reduction 7-10% 重量减少7-10% 2. Machine size reduction from 1000 tons to 500
tons注塑机从1000吨降低到500吨 3. Cycle time savings of 25-40%循环时间节省25-40% 4. 200% improvement in fatigue resistance抗疲劳性
Solid
-
- 0.0075 0.0120 0.0180
MuCell® #1 8% 16% 0.0050 0.0025 0.0060
MuCell® #2 20% 30% 0.0060 0.0020 0.0030
Δ=A-B
Dim #1
A
Dim #2
B
Dim
#3
Cost Savings成本节省
Key MuCell® Objectives 使用MuCell®目标
–
整个系统包括模具,材料,机器和工艺间配合。
New Trends - More Systems Integration Autobar SLIM®
新趋势-更多的综合应用
自动贴标系统SLIM®
Mucell模具技术应用
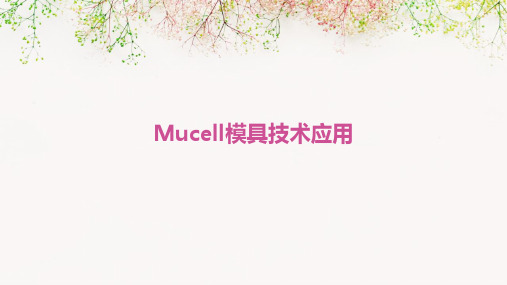
服务化转型,推动产业升级和经济发展。
THANK YOU
感谢聆听
Mucell模具技术应用
目
CONTENCT
录
• Mucell模具技术简介 • Mucell模具技术的工作原理 • Mucell模具技术的应用案例 • Mucell模具技术的未来发展 • 结论
01
Mucell模具技术简介
Mucell技术的起源和背景
Mucell技术起源于德国,最初是为了解决传统注塑模具生产中存 在的成本高、周期长等问题而研发的。
汽车制造行业的应用
汽车零部件制造
Mucell模具技术可用于制造汽 车零部件,如发动机罩、车门 、翼子板等,提高生产效率, 降低成本。
轻量化设计
通过Mucell模具技术,可以实 现汽车零部件的轻量化设计, 从而提高燃油经济性和减少排 放。
快速原型制作
Mucell模具技术可用于快速原 型制作,缩短产品开发周期, 加快新车型的上市速度。
选择高质量的材料,如不锈钢、铝合金等,以确 保模具的耐用性和精度。
加工与制造
将设计好的模具进行加工和制造,确保每个部分 符合设计要求。
设计与建模
根据产品需求进行模具设计,并使用CAD软件进 行建模。
检测与调试
完成制造后,对模具进行检测和调试,确保其正 常工作。
Mucell模具的工作原理
80%
注塑成型
05
结论
Mucell模具技术的价值和意义
高效生产
Mucell模具技术能够实现快速、 准确地生产出高质量的产品,提
高生产效率,降低生产成本。
定制化生产
Mucell模具技术可以根据客户需 求进行定制化生产,满足不同客 户的需求,提高产品的附加值和
高质量低成本的MuCell微发泡注塑成形技术
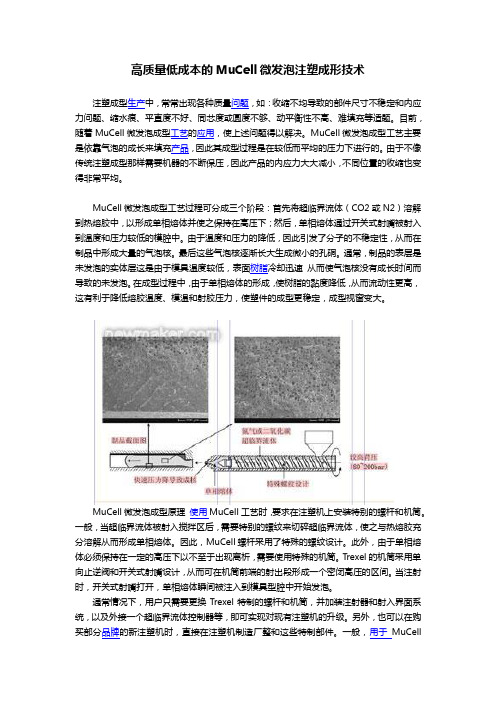
高质量低成本的MuCell微发泡注塑成形技术注塑成型生产中,常常出现各种质量问题,如:收缩不均导致的部件尺寸不稳定和内应力问题、缩水痕、平直度不好、同芯度或圆度不够、动平衡性不高、难填充等适题。
目前,随着MuCell微发泡成型工艺的应用,使上述问题得以解决。
MuCell微发泡成型工艺主要是依靠气泡的成长来填充产品,因此其成型过程是在较低而平均的压力下进行的。
由于不像传统注塑成型那样需要机器的不断保压,因此产品的内应力大大减小,不同位置的收缩也变得非常平均。
MuCell微发泡成型工艺过程可分成三个阶段:首先将超临界流体(CO2或N2)溶解到热熔胶中,以形成单相熔体并使之保持在高压下;然后,单相熔体通过开关式射嘴被射入到温度和压力较低的模腔中。
由于温度和压力的降低,因此引发了分子的不稳定性,从而在制品中形成大量的气泡核。
最后这些气泡核逐渐长大生成微小的孔硐。
通常,制品的表层是未发泡的实体层这是由于模具温度较低,表面树脂冷却迅速从而使气泡核没有成长时间而导致的未发泡。
在成型过程中,由于单相熔体的形成,使树脂的黏度降低,从而流动性更高,这有利于降低熔胶温度、模温和射胶压力,使塑件的成型更稳定,成型视窗变大。
MuCell微发泡成型原理使用MuCell工艺时,要求在注塑机上安装特别的螺杆和机筒。
一般,当超临界流体被射入搅拌区后,需要特别的螺纹来切碎超临界流体,使之与热熔胶充分溶解从而形成单相熔体。
因此,MuCell螺杆采用了特殊的螺纹设计。
此外,由于单相熔体必须保持在一定的高压下以不至于出现离析,需要使用特殊的机筒。
Trexel的机筒采用单向止逆阀和开关式射嘴设计,从而可在机筒前端的射出段形成一个密闭高压的区间。
当注射时,开关式射嘴打开,单相熔体瞬间被注入到模具型腔中开始发泡。
通常情况下,用户只需要更换Trexel特制的螺杆和机筒,并加装注射器和射入界面系统,以及外接一个超临界流体控制器等,即可实现对现有注塑机的升级。
高质量低成本的MuCell微发泡注塑成形技术
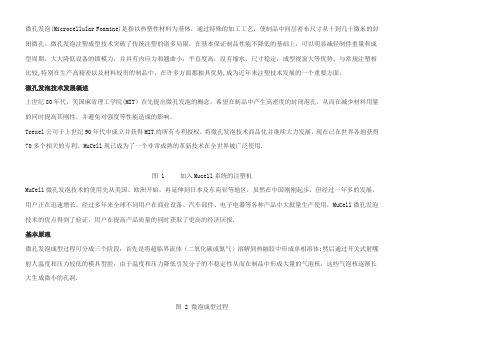
微孔发泡(Microcellular Foamine)是指以热塑性材料为基体,通过特殊的加工工艺,使制品中间层密布尺寸从十到几十微米的封闭微孔。
微孔发泡注塑成型技术突破了传统注塑的诸多局限,在基本保证制品性能不降低的基础上,可以明显减轻制件重量和成型周期,大大降低设备的锁模力,并具有内应力和翘曲小,平直度高,没有缩水,尺寸稳定,成型视窗大等优势。
与常规注塑相比较,特别在生产高精密以及材料较贵的制品中,在许多方面都独具优势,成为近年来注塑技术发展的一个重要方面。
微孔发泡技术发展概述上世纪80年代,美国麻省理工学院(MIT)首先提出微孔发泡的概念,希望在制品中产生高密度的封闭泡孔,从而在减少材料用量的同时提高其刚性,并避免对强度等性能造成的影响。
Trexel公司于上世纪90年代中成立并获得MIT的所有专利授权,将微孔发泡技术商品化并继续大力发展,现在已在世界各地获得70多个相关的专利。
MuCell现已成为了一个非常成熟的革新技术在全世界被广泛使用.图 1 加入Mucell系统的注塑机MuCell微孔发泡技术的使用先从美国、欧洲开始,再延伸到日本及东南亚等地区,虽然在中国刚刚起步,但经过一年多的发展,用户正在迅速增长。
经过多年来全球不同用户在商业设备、汽车部件、电子电器等各种产品中大批量生产使用,MuCell微孔发泡技术的优点得到了验证,用户在提高产品质量的同时获取了更高的经济回报.基本原理微孔发泡成型过程可分成三个阶段:首先是将超临界流体(二氧化碳或氮气)溶解到热融胶中形成单相溶体;然后通过开关式射嘴射人温度和压力较低的模具型腔,由于温度和压力降低引发分子的不稳定性从而在制品中形成大量的气泡核,这些气泡核逐渐长大生成微小的孔洞.图 2 微泡成型过程发泡后的制品横切面放大图如下,我们从中可以明显看到表层还是未发泡的实体层,这是由于模具温度较低,表面树脂冷却迅速,细胞核没有成长的时间,所以还是未发泡的实体。
Mucell微细发泡射出系统

力
57%) 4% )
• 4% weight reduction ( 量
Reduced Flash, Reduced Part Stress, Longer Clamp Tonnage Machines ( , 力, ,降 力)
Tool Life, Reduced
Applications (
Best(
16
Lower Cavity Pressure (降
Solid( ) MuCell( )
Peak CP = 1045 bar
力)
Peak CP = 448 bar
Screw position
• Cavity pressure reduced 57% (
Data Com Connector 30% Glass filled PBT
•
)
)
)
Limitation(
Foamable are almost all thermoplastic materials ( 料) High dimensional requirements – dimension, warpage, flatness, sink mark ( 度 — , , 度, ) Parts with high molded-in stress ( 力) High flow path – wall length/thickness ratio ( 流 度— 度/ ) Wall thickness of <3mm ( 3mm) Parts with high flash(零 )
)
Faster Product Release
)
)
Cost Reduction ( 省 )
(更
MuCell 基本原理
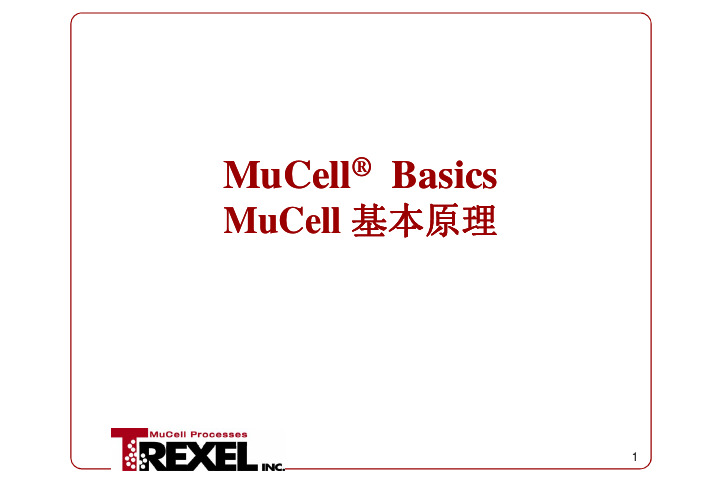
MuCell®Basics M C ll B i MuCell基本原理What is What is MuCell MuCell 什么是MuCell•MuCell is a foaming technology是一种发泡技术MuCell 是种发泡技术–Putting small cells into a thinwall plastic part–把微细胞加进薄壁塑胶零件之内•Primarily using nitrogen as thefoaming agent•主要以氮气为发泡剂–Sometimes carbon dioxide–有时会用到二氧化碳有时会用到氧化碳(CO2)Why•Why better cell structure with MuCellWhyWhy MuCellMuCell为什么MuCell能做到较佳之细胞结构–Direct introduction of physical foaming agent allows for higher level of actual foaming agent直接加入物理性发泡剂,以可容许较大量发泡剂被添进•Nitrogen or carbon dioxide或氧化碳•氮气(N2)或二氧化碳(CO2)–Higher level of foaming agent creates more cell sites 较大量发泡剂产生更多细胞较大量发泡剂产更多细胞–Cells grow uniformly keeping them small细胞可均匀地生长以及保持细小H d thi h•How does this happen如何做到Gas management equipment–气体管理设备•Equipment based technology 设备为本之技术-SCF Delivery SystemSCF传输系统–MIK (MuCell Interface Kit)MIK(MuCell 介面装置)()–Screw/Barrel Assembly螺杆/料管组合•Basic Steps•基本步骤–Melt plastic prior to SCF injection在注入SCF之前把塑料熔掉–Inject SCF during screw rotation在螺杆转动期间注入SCF–Dissolve SCF into the polymer melt (single phase Di l SCF i t th l lt(i l hsolution)把溶塑料熔融之中(单相熔体)SCF溶入塑料熔融之中单一相熔体)Inject SCFDissolve SCF注入SCF溶合Melt Polymer溶解塑胶料•Dissolving SCF溶解SCF–Screw flights break up SCF stream螺纹把SCF 切碎–Mixing flights cause SCF to break into smaller bubbles and then dissolve螺杆混合段把SCF 切成更微细气泡,以及把SCF 溶解于塑料中Inject SCF Di l SCF Inject SCF Dissolve SCF Dissolve SCF Diffusion注入SCF溶解SCFDiffusion Complete Polymer +SCF 聚合物•Once the SCF is dissolved into the molten polymer it must be kept under pressure一旦SCF溶入聚合物熔融,须确保料筒内压力得以维持•In the molding machine this meansI th ldi hi thi在注塑机台上是指–Screw position control螺杆位置控制–Shutoff Nozzle封闭式射咀•In the mold在模具上–Valve gates for hot runner molds热流道模具之针阀式浇口Foaming in the MoldLow pressure in the mold cause SCF to form 在模腔内发泡•cells模腔内之低压令SCF 产生细胞Time 时间C ll til th t i l f th ld •Cells grow until the material freezes or the mold cavity is full细胞不断地成生.持续到物料凝固或模腔被填满Controlling Foaming•Controlling Cell Size and the number of cells细胞尺寸以及数量之控股控制发泡–Filler level and type填充物份量(%)以及种类•Glass is preferred玻璃纤维较佳–Correct SCF level合适SCF 用量•Higher SCF creates more cells更高的SCF 产生更多细胞-Always a limit to how much SCF对每种物料,有不同的SCF 用量上限R t f d (t ll d i il b i j ti –Rate of pressure drop (controlled primarily by injection speed)压降的速率(主要由注射速度控制)•Shaking a bottle of soda results in more foaming 如摇晃一瓶苏打水导致更多泡沫。
毕业设计论文塑料注射成型
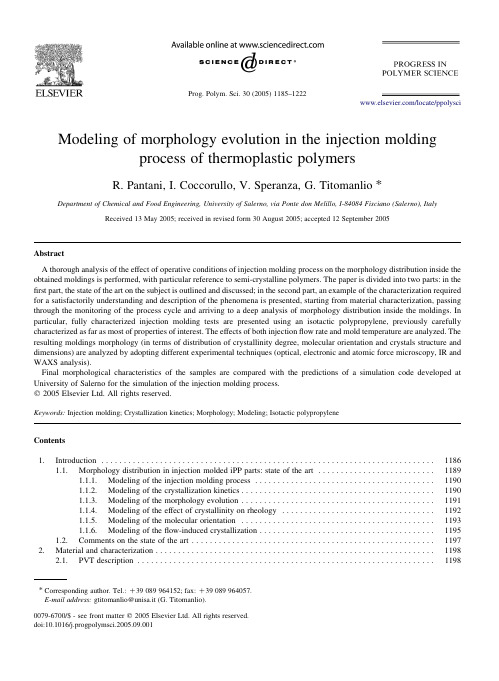
Modeling of morphology evolution in the injection moldingprocess of thermoplastic polymersR.Pantani,I.Coccorullo,V.Speranza,G.Titomanlio* Department of Chemical and Food Engineering,University of Salerno,via Ponte don Melillo,I-84084Fisciano(Salerno),Italy Received13May2005;received in revised form30August2005;accepted12September2005AbstractA thorough analysis of the effect of operative conditions of injection molding process on the morphology distribution inside the obtained moldings is performed,with particular reference to semi-crystalline polymers.The paper is divided into two parts:in the first part,the state of the art on the subject is outlined and discussed;in the second part,an example of the characterization required for a satisfactorily understanding and description of the phenomena is presented,starting from material characterization,passing through the monitoring of the process cycle and arriving to a deep analysis of morphology distribution inside the moldings.In particular,fully characterized injection molding tests are presented using an isotactic polypropylene,previously carefully characterized as far as most of properties of interest.The effects of both injectionflow rate and mold temperature are analyzed.The resulting moldings morphology(in terms of distribution of crystallinity degree,molecular orientation and crystals structure and dimensions)are analyzed by adopting different experimental techniques(optical,electronic and atomic force microscopy,IR and WAXS analysis).Final morphological characteristics of the samples are compared with the predictions of a simulation code developed at University of Salerno for the simulation of the injection molding process.q2005Elsevier Ltd.All rights reserved.Keywords:Injection molding;Crystallization kinetics;Morphology;Modeling;Isotactic polypropyleneContents1.Introduction (1186)1.1.Morphology distribution in injection molded iPP parts:state of the art (1189)1.1.1.Modeling of the injection molding process (1190)1.1.2.Modeling of the crystallization kinetics (1190)1.1.3.Modeling of the morphology evolution (1191)1.1.4.Modeling of the effect of crystallinity on rheology (1192)1.1.5.Modeling of the molecular orientation (1193)1.1.6.Modeling of theflow-induced crystallization (1195)ments on the state of the art (1197)2.Material and characterization (1198)2.1.PVT description (1198)*Corresponding author.Tel.:C39089964152;fax:C39089964057.E-mail address:gtitomanlio@unisa.it(G.Titomanlio).2.2.Quiescent crystallization kinetics (1198)2.3.Viscosity (1199)2.4.Viscoelastic behavior (1200)3.Injection molding tests and analysis of the moldings (1200)3.1.Injection molding tests and sample preparation (1200)3.2.Microscopy (1202)3.2.1.Optical microscopy (1202)3.2.2.SEM and AFM analysis (1202)3.3.Distribution of crystallinity (1202)3.3.1.IR analysis (1202)3.3.2.X-ray analysis (1203)3.4.Distribution of molecular orientation (1203)4.Analysis of experimental results (1203)4.1.Injection molding tests (1203)4.2.Morphology distribution along thickness direction (1204)4.2.1.Optical microscopy (1204)4.2.2.SEM and AFM analysis (1204)4.3.Morphology distribution alongflow direction (1208)4.4.Distribution of crystallinity (1210)4.4.1.Distribution of crystallinity along thickness direction (1210)4.4.2.Crystallinity distribution alongflow direction (1212)4.5.Distribution of molecular orientation (1212)4.5.1.Orientation along thickness direction (1212)4.5.2.Orientation alongflow direction (1213)4.5.3.Direction of orientation (1214)5.Simulation (1214)5.1.Pressure curves (1215)5.2.Morphology distribution (1215)5.3.Molecular orientation (1216)5.3.1.Molecular orientation distribution along thickness direction (1216)5.3.2.Molecular orientation distribution alongflow direction (1216)5.3.3.Direction of orientation (1217)5.4.Crystallinity distribution (1217)6.Conclusions (1217)References (1219)1.IntroductionInjection molding is one of the most widely employed methods for manufacturing polymeric products.Three main steps are recognized in the molding:filling,packing/holding and cooling.During thefilling stage,a hot polymer melt rapidlyfills a cold mold reproducing a cavity of the desired product shape. During the packing/holding stage,the pressure is raised and extra material is forced into the mold to compensate for the effects that both temperature decrease and crystallinity development determine on density during solidification.The cooling stage starts at the solidification of a thin section at cavity entrance (gate),starting from that instant no more material can enter or exit from the mold impression and holding pressure can be released.When the solid layer on the mold surface reaches a thickness sufficient to assure required rigidity,the product is ejected from the mold.Due to the thermomechanical history experienced by the polymer during processing,macromolecules in injection-molded objects present a local order.This order is referred to as‘morphology’which literally means‘the study of the form’where form stands for the shape and arrangement of parts of the object.When referred to polymers,the word morphology is adopted to indicate:–crystallinity,which is the relative volume occupied by each of the crystalline phases,including mesophases;–dimensions,shape,distribution and orientation of the crystallites;–orientation of amorphous phase.R.Pantani et al./Prog.Polym.Sci.30(2005)1185–1222 1186R.Pantani et al./Prog.Polym.Sci.30(2005)1185–12221187Apart from the scientific interest in understandingthe mechanisms leading to different order levels inside a polymer,the great technological importance of morphology relies on the fact that polymer character-istics (above all mechanical,but also optical,electrical,transport and chemical)are to a great extent affected by morphology.For instance,crystallinity has a pro-nounced effect on the mechanical properties of the bulk material since crystals are generally stiffer than amorphous material,and also orientation induces anisotropy and other changes in mechanical properties.In this work,a thorough analysis of the effect of injection molding operative conditions on morphology distribution in moldings with particular reference to crystalline materials is performed.The aim of the paper is twofold:first,to outline the state of the art on the subject;second,to present an example of the characterization required for asatisfactorilyR.Pantani et al./Prog.Polym.Sci.30(2005)1185–12221188understanding and description of the phenomena, starting from material description,passing through the monitoring of the process cycle and arriving to a deep analysis of morphology distribution inside the mold-ings.To these purposes,fully characterized injection molding tests were performed using an isotactic polypropylene,previously carefully characterized as far as most of properties of interest,in particular quiescent nucleation density,spherulitic growth rate and rheological properties(viscosity and relaxation time)were determined.The resulting moldings mor-phology(in terms of distribution of crystallinity degree, molecular orientation and crystals structure and dimensions)was analyzed by adopting different experimental techniques(optical,electronic and atomic force microscopy,IR and WAXS analysis).Final morphological characteristics of the samples were compared with the predictions of a simulation code developed at University of Salerno for the simulation of the injection molding process.The effects of both injectionflow rate and mold temperature were analyzed.1.1.Morphology distribution in injection molded iPP parts:state of the artFrom many experimental observations,it is shown that a highly oriented lamellar crystallite microstructure, usually referred to as‘skin layer’forms close to the surface of injection molded articles of semi-crystalline polymers.Far from the wall,the melt is allowed to crystallize three dimensionally to form spherulitic structures.Relative dimensions and morphology of both skin and core layers are dependent on local thermo-mechanical history,which is characterized on the surface by high stress levels,decreasing to very small values toward the core region.As a result,the skin and the core reveal distinct characteristics across the thickness and also along theflow path[1].Structural and morphological characterization of the injection molded polypropylene has attracted the interest of researchers in the past three decades.In the early seventies,Kantz et al.[2]studied the morphology of injection molded iPP tensile bars by using optical microscopy and X-ray diffraction.The microscopic results revealed the presence of three distinct crystalline zones on the cross-section:a highly oriented non-spherulitic skin;a shear zone with molecular chains oriented essentially parallel to the injection direction;a spherulitic core with essentially no preferred orientation.The X-ray diffraction studies indicated that the skin layer contains biaxially oriented crystallites due to the biaxial extensionalflow at theflow front.A similar multilayered morphology was also reported by Menges et al.[3].Later on,Fujiyama et al.[4] investigated the skin–core morphology of injection molded iPP samples using X-ray Small and Wide Angle Scattering techniques,and suggested that the shear region contains shish–kebab structures.The same shish–kebab structure was observed by Wenig and Herzog in the shear region of their molded samples[5].A similar investigation was conducted by Titomanlio and co-workers[6],who analyzed the morphology distribution in injection moldings of iPP. They observed a skin–core morphology distribution with an isotropic spherulitic core,a skin layer characterized by afine crystalline structure and an intermediate layer appearing as a dark band in crossed polarized light,this layer being characterized by high crystallinity.Kalay and Bevis[7]pointed out that,although iPP crystallizes essentially in the a-form,a small amount of b-form can be found in the skin layer and in the shear region.The amount of b-form was found to increase by effect of high shear rates[8].A wide analysis on the effect of processing conditions on the morphology of injection molded iPP was conducted by Viana et al.[9]and,more recently, by Mendoza et al.[10].In particular,Mendoza et al. report that the highest level of crystallinity orientation is found inside the shear zone and that a high level of orientation was also found in the skin layer,with an orientation angle tilted toward the core.It is rather difficult to theoretically establish the relationship between the observed microstructure and processing conditions.Indeed,a model of the injection molding process able to predict morphology distribution in thefinal samples is not yet available,even if it would be of enormous strategic importance.This is mainly because a complete understanding of crystallization kinetics in processing conditions(high cooling rates and pressures,strong and complexflowfields)has not yet been reached.In this section,the most relevant aspects for process modeling and morphology development are identified. In particular,a successful path leading to a reliable description of morphology evolution during polymer processing should necessarily pass through:–a good description of morphology evolution under quiescent conditions(accounting all competing crystallization processes),including the range of cooling rates characteristic of processing operations (from1to10008C/s);R.Pantani et al./Prog.Polym.Sci.30(2005)1185–12221189–a description capturing the main features of melt morphology(orientation and stretch)evolution under processing conditions;–a good coupling of the two(quiescent crystallization and orientation)in order to capture the effect of crystallinity on viscosity and the effect offlow on crystallization kinetics.The points listed above outline the strategy to be followed in order to achieve the basic understanding for a satisfactory description of morphology evolution during all polymer processing operations.In the following,the state of art for each of those points will be analyzed in a dedicated section.1.1.1.Modeling of the injection molding processThefirst step in the prediction of the morphology distribution within injection moldings is obviously the thermo-mechanical simulation of the process.Much of the efforts in the past were focused on the prediction of pressure and temperature evolution during the process and on the prediction of the melt front advancement [11–15].The simulation of injection molding involves the simultaneous solution of the mass,energy and momentum balance equations.Thefluid is non-New-tonian(and viscoelastic)with all parameters dependent upon temperature,pressure,crystallinity,which are all function of pressibility cannot be neglected as theflow during the packing/holding step is determined by density changes due to temperature, pressure and crystallinity evolution.Indeed,apart from some attempts to introduce a full 3D approach[16–19],the analysis is currently still often restricted to the Hele–Shaw(or thinfilm) approximation,which is warranted by the fact that most injection molded parts have the characteristic of being thin.Furthermore,it is recognized that the viscoelastic behavior of the polymer only marginally influences theflow kinematics[20–22]thus the melt is normally considered as a non-Newtonian viscousfluid for the description of pressure and velocity gradients evolution.Some examples of adopting a viscoelastic constitutive equation in the momentum balance equations are found in the literature[23],but the improvements in accuracy do not justify a considerable extension of computational effort.It has to be mentioned that the analysis of some features of kinematics and temperature gradients affecting the description of morphology need a more accurate description with respect to the analysis of pressure distributions.Some aspects of the process which were often neglected and may have a critical importance are the description of the heat transfer at polymer–mold interface[24–26]and of the effect of mold deformation[24,27,28].Another aspect of particular interest to the develop-ment of morphology is the fountainflow[29–32], which is often neglected being restricted to a rather small region at theflow front and close to the mold walls.1.1.2.Modeling of the crystallization kineticsIt is obvious that the description of crystallization kinetics is necessary if thefinal morphology of the molded object wants to be described.Also,the development of a crystalline degree during the process influences the evolution of all material properties like density and,above all,viscosity(see below).Further-more,crystallization kinetics enters explicitly in the generation term of the energy balance,through the latent heat of crystallization[26,33].It is therefore clear that the crystallinity degree is not only a result of simulation but also(and above all)a phenomenon to be kept into account in each step of process modeling.In spite of its dramatic influence on the process,the efforts to simulate the injection molding of semi-crystalline polymers are crude in most of the commercial software for processing simulation and rather scarce in the fleur and Kamal[34],Papatanasiu[35], Titomanlio et al.[15],Han and Wang[36],Ito et al.[37],Manzione[38],Guo and Isayev[26],and Hieber [25]adopted the following equation(Kolmogoroff–Avrami–Evans,KAE)to predict the development of crystallinityd xd tZð1K xÞd d cd t(1)where x is the relative degree of crystallization;d c is the undisturbed volume fraction of the crystals(if no impingement would occur).A significant improvement in the prediction of crystallinity development was introduced by Titoman-lio and co-workers[39]who kept into account the possibility of the formation of different crystalline phases.This was done by assuming a parallel of several non-interacting kinetic processes competing for the available amorphous volume.The evolution of each phase can thus be described byd x id tZð1K xÞd d c id t(2)where the subscript i stands for a particular phase,x i is the relative degree of crystallization,x ZPix i and d c iR.Pantani et al./Prog.Polym.Sci.30(2005)1185–1222 1190is the expectancy of volume fraction of each phase if no impingement would occur.Eq.(2)assumes that,for each phase,the probability of the fraction increase of a single crystalline phase is simply the product of the rate of growth of the corresponding undisturbed volume fraction and of the amount of available amorphous fraction.By summing up the phase evolution equations of all phases(Eq.(2))over the index i,and solving the resulting differential equation,one simply obtainsxðtÞZ1K exp½K d cðtÞ (3)where d c Z Pid c i and Eq.(1)is recovered.It was shown by Coccorullo et al.[40]with reference to an iPP,that the description of the kinetic competition between phases is crucial to a reliable prediction of solidified structures:indeed,it is not possible to describe iPP crystallization kinetics in the range of cooling rates of interest for processing(i.e.up to several hundreds of8C/s)if the mesomorphic phase is neglected:in the cooling rate range10–1008C/s, spherulite crystals in the a-phase are overcome by the formation of the mesophase.Furthermore,it has been found that in some conditions(mainly at pressures higher than100MPa,and low cooling rates),the g-phase can also form[41].In spite of this,the presence of different crystalline phases is usually neglected in the literature,essentially because the range of cooling rates investigated for characterization falls in the DSC range (well lower than typical cooling rates of interest for the process)and only one crystalline phase is formed for iPP at low cooling rates.It has to be noticed that for iPP,which presents a T g well lower than ambient temperature,high values of crystallinity degree are always found in solids which passed through ambient temperature,and the cooling rate can only determine which crystalline phase forms, roughly a-phase at low cooling rates(below about 508C/s)and mesomorphic phase at higher cooling rates.The most widespread approach to the description of kinetic constant is the isokinetic approach introduced by Nakamura et al.According to this model,d c in Eq.(1)is calculated asd cðtÞZ ln2ðt0KðTðsÞÞd s2 435n(4)where K is the kinetic constant and n is the so-called Avrami index.When introduced as in Eq.(4),the reciprocal of the kinetic constant is a characteristic time for crystallization,namely the crystallization half-time, t05.If a polymer is cooled through the crystallization temperature,crystallization takes place at the tempera-ture at which crystallization half-time is of the order of characteristic cooling time t q defined ast q Z D T=q(5) where q is the cooling rate and D T is a temperature interval over which the crystallization kinetic constant changes of at least one order of magnitude.The temperature dependence of the kinetic constant is modeled using some analytical function which,in the simplest approach,is described by a Gaussian shaped curve:KðTÞZ K0exp K4ln2ðT K T maxÞ2D2(6)The following Hoffman–Lauritzen expression[42] is also commonly adopted:K½TðtÞ Z K0exp KUÃR$ðTðtÞK T NÞ!exp KKÃ$ðTðtÞC T mÞ2TðtÞ2$ðT m K TðtÞÞð7ÞBoth equations describe a bell shaped curve with a maximum which for Eq.(6)is located at T Z T max and for Eq.(7)lies at a temperature between T m(the melting temperature)and T N(which is classically assumed to be 308C below the glass transition temperature).Accord-ing to Eq.(7),the kinetic constant is exactly zero at T Z T m and at T Z T N,whereas Eq.(6)describes a reduction of several orders of magnitude when the temperature departs from T max of a value higher than2D.It is worth mentioning that only three parameters are needed for Eq.(6),whereas Eq.(7)needs the definition offive parameters.Some authors[43,44]couple the above equations with the so-called‘induction time’,which can be defined as the time the crystallization process starts, when the temperature is below the equilibrium melting temperature.It is normally described as[45]Dt indDtZðT0m K TÞat m(8)where t m,T0m and a are material constants.It should be mentioned that it has been found[46,47]that there is no need to explicitly incorporate an induction time when the modeling is based upon the KAE equation(Eq.(1)).1.1.3.Modeling of the morphology evolutionDespite of the fact that the approaches based on Eq.(4)do represent a significant step toward the descriptionR.Pantani et al./Prog.Polym.Sci.30(2005)1185–12221191of morphology,it has often been pointed out in the literature that the isokinetic approach on which Nakamura’s equation (Eq.(4))is based does not describe details of structure formation [48].For instance,the well-known experience that,with many polymers,the number of spherulites in the final solid sample increases strongly with increasing cooling rate,is indeed not taken into account by this approach.Furthermore,Eq.(4)describes an increase of crystal-linity (at constant temperature)depending only on the current value of crystallinity degree itself,whereas it is expected that the crystallization rate should depend also on the number of crystalline entities present in the material.These limits are overcome by considering the crystallization phenomenon as the consequence of nucleation and growth.Kolmogoroff’s model [49],which describes crystallinity evolution accounting of the number of nuclei per unit volume and spherulitic growth rate can then be applied.In this case,d c in Eq.(1)is described asd ðt ÞZ C m ðt 0d N ðs Þd s$ðt sG ðu Þd u 2435nd s (9)where C m is a shape factor (C 3Z 4/3p ,for spherical growth),G (T (t ))is the linear growth rate,and N (T (t ))is the nucleation density.The following Hoffman–Lauritzen expression is normally adopted for the growth rateG ½T ðt Þ Z G 0exp KUR $ðT ðt ÞK T N Þ!exp K K g $ðT ðt ÞC T m Þ2T ðt Þ2$ðT m K T ðt ÞÞð10ÞEqs.(7)and (10)have the same form,however the values of the constants are different.The nucleation mechanism can be either homo-geneous or heterogeneous.In the case of heterogeneous nucleation,two equations are reported in the literature,both describing the nucleation density as a function of temperature [37,50]:N ðT ðt ÞÞZ N 0exp ½j $ðT m K T ðt ÞÞ (11)N ðT ðt ÞÞZ N 0exp K 3$T mT ðt ÞðT m K T ðt ÞÞ(12)In the case of homogeneous nucleation,the nucleation rate rather than the nucleation density is function of temperature,and a Hoffman–Lauritzen expression isadoptedd N ðT ðt ÞÞd t Z N 0exp K C 1ðT ðt ÞK T N Þ!exp KC 2$ðT ðt ÞC T m ÞT ðt Þ$ðT m K T ðt ÞÞð13ÞConcentration of nucleating particles is usually quite significant in commercial polymers,and thus hetero-geneous nucleation becomes the dominant mechanism.When Kolmogoroff’s approach is followed,the number N a of active nuclei at the end of the crystal-lization process can be calculated as [48]N a ;final Zðt final 0d N ½T ðs Þd sð1K x ðs ÞÞd s (14)and the average dimension of crystalline structures can be attained by geometrical considerations.Pantani et al.[51]and Zuidema et al.[22]exploited this method to describe the distribution of crystallinity and the final average radius of the spherulites in injection moldings of polypropylene;in particular,they adopted the following equationR Z ffiffiffiffiffiffiffiffiffiffiffiffiffiffiffiffiffiffiffi3x a ;final 4p N a ;final 3s (15)A different approach is also present in the literature,somehow halfway between Nakamura’s and Kolmo-goroff’s models:the growth rate (G )and the kinetic constant (K )are described independently,and the number of active nuclei (and consequently the average dimensions of crystalline entities)can be obtained by coupling Eqs.(4)and (9)asN a ðT ÞZ 3ln 24p K ðT ÞG ðT Þ 3(16)where heterogeneous nucleation and spherical growth is assumed (Avrami’s index Z 3).Guo et al.[43]adopted this approach to describe the dimensions of spherulites in injection moldings of polypropylene.1.1.4.Modeling of the effect of crystallinity on rheology As mentioned above,crystallization has a dramatic influence on material viscosity.This phenomenon must obviously be taken into account and,indeed,the solidification of a semi-crystalline material is essen-tially caused by crystallization rather than by tempera-ture in normal processing conditions.Despite of the importance of the subject,the relevant literature on the effect of crystallinity on viscosity isR.Pantani et al./Prog.Polym.Sci.30(2005)1185–12221192rather scarce.This might be due to the difficulties in measuring simultaneously rheological properties and crystallinity evolution during the same tests.Apart from some attempts to obtain simultaneous measure-ments of crystallinity and viscosity by special setups [52,53],more often viscosity and crystallinity are measured during separate tests having the same thermal history,thus greatly simplifying the experimental approach.Nevertheless,very few works can be retrieved in the literature in which(shear or complex) viscosity can be somehow linked to a crystallinity development.This is the case of Winter and co-workers [54],Vleeshouwers and Meijer[55](crystallinity evolution can be drawn from Swartjes[56]),Boutahar et al.[57],Titomanlio et al.[15],Han and Wang[36], Floudas et al.[58],Wassner and Maier[59],Pantani et al.[60],Pogodina et al.[61],Acierno and Grizzuti[62].All the authors essentially agree that melt viscosity experiences an abrupt increase when crystallinity degree reaches a certain‘critical’value,x c[15]. However,little agreement is found in the literature on the value of this critical crystallinity degree:assuming that x c is reached when the viscosity increases of one order of magnitude with respect to the molten state,it is found in the literature that,for iPP,x c ranges from a value of a few percent[15,62,60,58]up to values of20–30%[58,61]or even higher than40%[59,54,57].Some studies are also reported on the secondary effects of relevant variables such as temperature or shear rate(or frequency)on the dependence of crystallinity on viscosity.As for the effect of temperature,Titomanlio[15]found for an iPP that the increase of viscosity for the same crystallinity degree was higher at lower temperatures,whereas Winter[63] reports the opposite trend for a thermoplastic elasto-meric polypropylene.As for the effect of shear rate,a general agreement is found in the literature that the increase of viscosity for the same crystallinity degree is lower at higher deformation rates[62,61,57].Essentially,the equations adopted to describe the effect of crystallinity on viscosity of polymers can be grouped into two main categories:–equations based on suspensions theories(for a review,see[64]or[65]);–empirical equations.Some of the equations adopted in the literature with regard to polymer processing are summarized in Table1.Apart from Eq.(17)adopted by Katayama and Yoon [66],all equations predict a sharp increase of viscosity on increasing crystallinity,sometimes reaching infinite (Eqs.(18)and(21)).All authors consider that the relevant variable is the volume occupied by crystalline entities(i.e.x),even if the dimensions of the crystals should reasonably have an effect.1.1.5.Modeling of the molecular orientationOne of the most challenging problems to present day polymer science regards the reliable prediction of molecular orientation during transformation processes. Indeed,although pressure and velocity distribution during injection molding can be satisfactorily described by viscous models,details of the viscoelastic nature of the polymer need to be accounted for in the descriptionTable1List of the most used equations to describe the effect of crystallinity on viscosityEquation Author Derivation Parameters h=h0Z1C a0x(17)Katayama[66]Suspensions a Z99h=h0Z1=ðx K x cÞa0(18)Ziabicki[67]Empirical x c Z0.1h=h0Z1C a1expðK a2=x a3Þ(19)Titomanlio[15],also adopted byGuo[68]and Hieber[25]Empiricalh=h0Z expða1x a2Þ(20)Shimizu[69],also adopted byZuidema[22]and Hieber[25]Empiricalh=h0Z1Cðx=a1Þa2=ð1Kðx=a1Þa2Þ(21)Tanner[70]Empirical,basedon suspensionsa1Z0.44for compact crystallitesa1Z0.68for spherical crystallitesh=h0Z expða1x C a2x2Þ(22)Han[36]Empiricalh=h0Z1C a1x C a2x2(23)Tanner[71]Empirical a1Z0.54,a2Z4,x!0.4h=h0Zð1K x=a0ÞK2(24)Metzner[65],also adopted byTanner[70]Suspensions a Z0.68for smooth spheresR.Pantani et al./Prog.Polym.Sci.30(2005)1185–12221193。
MuCell微发泡注塑成型技术应用

打 印机 内部部 件 、 导纸 机 构 、 压 纸 卷 筒 、托 架 和 墨 辊 发动 机 罩 和动 力 系统 下 面 的部 件 、进 气 管 墨 粉 盒 复 印 机 电 气 /电 子部 件 、变 速 箱 、运 动部 件 采 暖 、通 风 、 空调 和 散 热系 统 部 件 、 风扇 护 罩 、 阀 门和 发动 机 外 壳 密 封 部 件 、 门板 、手 套 式操 作 箱 、扬 声 器 外 壳
胞 的 成 长 来 填 充 产 品 , 所 以 是 在 较 低
射 嘴打 开 ,单 相 融 体 瞬 间注 进
模 具 型 腔开 始 发 泡 。
Mu l基 本 原 理 CeI
用 户可 以在 现有 注 塑 机 上
表 1 M C l微 发泡 技术 典 型 应 用领 域 u el
商 用 设 备 汽 车 电 气 /电 子
一
Trx l的 机 筒 有 单 向 止 逆 阀 ee
和开 关 式射 嘴 设 计 从 而在 机 筒 前真 个 射 出段 形 成 一个 密 闭高 压 的 区间 。 当注射 时 ,开 关 式
些 常 用 的产 品 ,当然 在 其 他 行 业 的
不 同领 域 也有 很 多用 户 成 功 应 用 了 这 种技术。 MuC l 微 发 泡 成 型 主 要 是 靠 细 el
为 一 种 非 常 成 熟 的 注 塑 成 型 革 新 技 术 ,在 全 世 界被 广 泛 使 用 。其 使 用 先 从 美 国 、欧 洲开 始 ,再 延 伸 到 日本 及
东 南 亚 等 地 区 ;尽 管 在 中 国 刚 刚 起
性 从而在制 品中形成大量 的气泡核 , 最 后 这 些 气 泡核 逐 渐 长大 天 生 微小 的
在 填 充 过 程 中模 具 温度 较 低 ,表 面 的 树 脂 冷 却 迅 速 ,细 胞 核 没 有 成 长 的 时 间所 以还未 发泡 。 使 用 Mu l 必 须 在 注 塑 机 上 装 Cel
微细发泡技术在塑料注塑件质量改善与减重之应用

【为制造而设计的准则、局限了设计自由性】
MuCell Injection Technology application
MuCell注塑技术的应用
1. MuCell Technology Introduction
MuCell技术介绍
2. Phase 1: Cost Saving
Quality Benefits 质量提升:
3、Design Freedom 设计更自由
MuCell Design Rules
• •
产品设计新准则
Cell growth by gas expansion replaces traditional pack phase 气体膨胀细胞变大取代传统保压 Design for function not for process
• • •
Product 产品: Water End Tank轼水箱 Material 材料: PA66/30GF Economic Benefits 经济价值:
•
• • • •
Cycle Reduction 周期减少: 20% Weight Reduction 重量减少: 20% Tonnage Reduction 吨位减少: 50% Improve warpage (60%) 减少变形(60%)
MuCell Design Rules
•
产品设计新准则
Rib to wall thicknesses ratios optimized for performance not sink mark elimination. Wall /Rib – thickness ratio 1:1 possible without sink mark. 筋壁厚比为产品性能优化而非缩水。加强筋厚度与塑件厚度之比可达
干细胞细胞膜仿生纳米材料-概念解析以及定义

干细胞细胞膜仿生纳米材料-概述说明以及解释1.引言1.1 概述引言是一篇文章中的重要组成部分,它用于引发读者的兴趣并介绍文章的主题。
本文的主题是干细胞细胞膜仿生纳米材料。
干细胞是一种具有自我更新和分化为多种细胞类型能力的特殊细胞。
细胞膜仿生纳米材料是通过模仿干细胞细胞膜结构和功能来制备的一种新型纳米材料。
干细胞细胞膜仿生纳米材料具有许多潜在的应用价值,可以在生物医学领域、药物传递系统和组织修复等方面发挥重要作用。
该材料具有许多优点,如良好的生物相容性、高度可控的形貌和组成,以及与细胞相似的生物功能。
在本文中,我们将首先介绍干细胞的定义和特点,包括干细胞的分类、来源和功能。
然后,我们将详细介绍细胞膜仿生纳米材料的概念和原理,包括干细胞细胞膜的复杂结构和功能。
接下来,我们将介绍一些常用的制备方法,包括自组装、脂质包裹和电化学方法等,并讨论它们的优缺点和应用领域。
最后,我们将展望干细胞细胞膜仿生纳米材料的应用前景,包括其在肿瘤治疗、神经退行性疾病治疗和再生医学中的潜在应用。
我们还将探讨未来发展方向,包括材料改性、多功能化以及与其他纳米材料的组合应用等。
通过本文的阅读,读者将对干细胞细胞膜仿生纳米材料有一个全面的了解,同时也能够了解其在生物医学领域中的前沿应用和未来发展方向。
文章的结构将按照上述大纲进行展开,以帮助读者更好地理解和掌握相关知识。
1.2 文章结构文章结构部分的内容可以按照以下方式编写:文章结构:本文将分为引言、正文和结论三个部分。
引言部分将概述干细胞细胞膜仿生纳米材料的研究背景和意义,介绍干细胞的定义和特点,并对文章的结构和目的进行简要说明。
正文部分将详细介绍干细胞细胞膜仿生纳米材料的概念和原理,包括细胞膜仿生纳米材料的定义、特点和应用前景。
同时,将介绍干细胞细胞膜仿生纳米材料的制备方法,包括常用的方法和新兴的研究进展。
结论部分将总结干细胞细胞膜仿生纳米材料的应用前景,并探讨未来的发展方向。
马尔文仪器助生物塑料工业优化生产

在 推 出了针 对可 追溯性 序 列化 管理 的 XMV喷码 影像 识别 系
上 海 外 高 桥 发 电有 限 责 任 公 司 是 以 上 海 外 高 桥 发 电厂 为 统 后 ,为 了 让 制 药 行 业 的 客 户 获 得 更 精 确 的 产 品 处 理 结 果 , 2M 基 础 组 建 而 成 的 公 司 。 上 海 外 高 桥 发 电厂 是 国 家 “ 五 ” 重 将 自动 检 重 秤 与 之 有 机 结 合 ,推 出 了 紧 凑 型 设 计 的 XS V 八 点 工 程 , 也 是 上 海 浦 东 第 一 批 十 大 基 础 设 施 工 程 投 资 最 多 的
天 力 公 司 完 成 ,设 备 年 利 用 为 7 6 小 时 , 产 线 能 力 ≥ l t l0 生 4/ h,有 效 容 积 为 5 0 ,采 用 先 进 、成 熟 、可 靠 的 技 术 ,装 置 0 m, 的服 务寿命 可达 3 0年 。
梅特 勒 一托利 多推 出新 型 自动 检 重秤
天力 公司 与上海 外高 桥 电力 股份 有 限公司 的首 次合 作得
到 了山东 省科学 院领导 的大 力支 持 。 生产 线坚 持 工艺简 捷 、 该 设 计 合 理 、技 术 先 进 、节 能 降 耗 、减 少 污 染 的 原 则 ,在 设 计 、
制造 、安装 、调 试 、试验 及检查 、 试运 等方 面均按 照标 准 由
方 面也 要求对 聚 合物 性能 进行 严格 控 制 。
N a u e 0r t r W kS的 研 究 专 家 J d Ra" a l称 赞 说 , e id l i
“ sn Ro a d和 TDAma x的卓越 性 能为我 们提 供 了 巨大 价值 ” , “ 我们使 用马 尔文 Ro a d毛 细管流 变仪 评估 添加剂 和分 子结 sn
木质素,聚乙二醇二缩水甘油醚,球磨
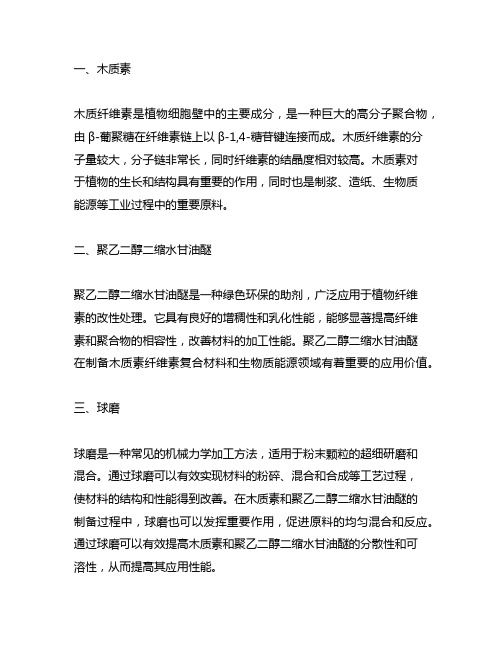
一、木质素木质纤维素是植物细胞壁中的主要成分,是一种巨大的高分子聚合物,由β-葡聚糖在纤维素链上以β-1,4-糖苷键连接而成。
木质纤维素的分子量较大,分子链非常长,同时纤维素的结晶度相对较高。
木质素对于植物的生长和结构具有重要的作用,同时也是制浆、造纸、生物质能源等工业过程中的重要原料。
二、聚乙二醇二缩水甘油醚聚乙二醇二缩水甘油醚是一种绿色环保的助剂,广泛应用于植物纤维素的改性处理。
它具有良好的增稠性和乳化性能,能够显著提高纤维素和聚合物的相容性,改善材料的加工性能。
聚乙二醇二缩水甘油醚在制备木质素纤维素复合材料和生物质能源领域有着重要的应用价值。
三、球磨球磨是一种常见的机械力学加工方法,适用于粉末颗粒的超细研磨和混合。
通过球磨可以有效实现材料的粉碎、混合和合成等工艺过程,使材料的结构和性能得到改善。
在木质素和聚乙二醇二缩水甘油醚的制备过程中,球磨也可以发挥重要作用,促进原料的均匀混合和反应。
通过球磨可以有效提高木质素和聚乙二醇二缩水甘油醚的分散性和可溶性,从而提高其应用性能。
四、综合应用结合以上三种原料和工艺方法,可以实现木质素和聚乙二醇二缩水甘油醚的混合改性。
在球磨的环境下,将木质素和聚乙二醇二缩水甘油醚进行均匀混合,并进行相应的反应处理。
随后,利用合适的工艺条件,使得木质素和聚乙二醇二缩水甘油醚充分反应,形成具有良好性能和稳定结构的复合材料。
通过相关工艺技术,制备出具有优异性能和广泛应用前景的木质素聚乙二醇二缩水甘油醚复合材料。
五、结语从木质素、聚乙二醇二缩水甘油醚和球磨这三个方面入手,可以更好地挖掘其在材料改性和生物质能源开发中的应用潜力。
通过合理的工艺设计和工艺优化,可以实现木质素和聚乙二醇二缩水甘油醚的混合改性,为生物质能源的高效利用和功能材料的研发提供有力支持。
希望在不久的将来,木质素聚乙二醇二缩水甘油醚复合材料能够更广泛地应用于各领域,推动材料科学和工程技术的发展。
六、木质素聚乙二醇二缩水甘油醚复合材料的应用木质素聚乙二醇二缩水甘油醚复合材料是一种具有广泛应用前景的新型功能材料。
聚研 Millipore液态芯片
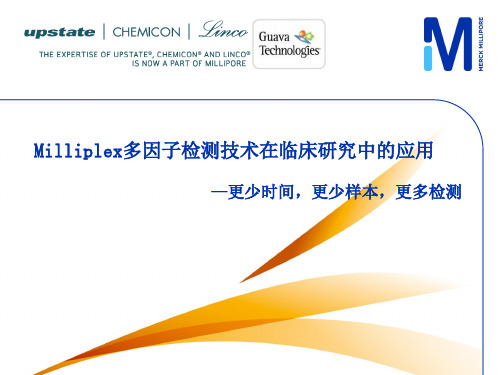
生物标志物筛选 — xMAP技术
使用2或3种荧光染料,通过调整染料之间的荧光比例,获得100或500 种不同的颜色
染色5.6mm的塑料微球/6.5mm磁球,获得100或500种不同颜色的微球
2 Dyes •Red •Infrared
3 Dyes •Red •Infrared •Orange Red
• 1997年,Clinical Chemistry杂志介绍Luminex技术,誉之为“真正的临床应用型 生物芯片”。
• 2001年,Luminex技术获美国FDA认证,成为首个,也是唯一得到美国FDA许可的用 于临床诊断的多指标检测技术。
• 2005年6月,基于Luminex技术的文章发表在《Nature》杂志上,标志着该技术在科 学研究领域得到的最高荣誉。
RANTES
IL-8
GRO
IL-9
IP-10
IL-10 IL-12p40 IL-12p70 IL-13 IL-15 IL-17 Eotaxin FGF-2 Fit-3 ligand IFNα2
MCP-1 MIP-1a MIP-1b sCD40L Fractalkine PDGF-AA PDGF-AB/BB TNFβ
Porcine Cytokine
Mouse Panels
Adipokine Bone CVD Cytokine Endocrine Gut Hormone Soluble Cytokine Receptor
xMAP技术在转化医学研究中的应用
疾病研究
疾病的早期发现与进程预测
•恶性肿瘤研究:组织脏器的早期癌变发现及肿瘤转移 •心血管疾病 •内分泌疾病 •神经退行性疾病:阿尔兹海默症 •自身免疫疾病:败血症、多发性硬化、类风湿关节炎
德国以纳米微粒为原料制成多层生物降解塑膜
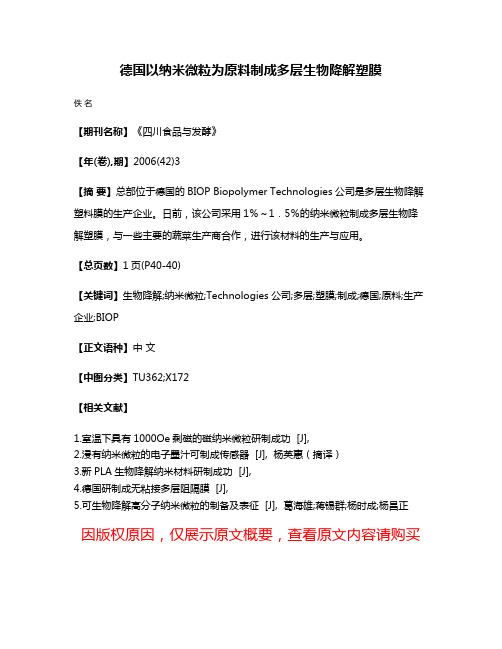
德国以纳米微粒为原料制成多层生物降解塑膜
佚名
【期刊名称】《四川食品与发酵》
【年(卷),期】2006(42)3
【摘要】总部位于德国的BIOP Biopolymer Technologies公司是多层生物降解塑料膜的生产企业。
日前,该公司采用1%~1.5%的纳米微粒制成多层生物降解塑膜,与一些主要的蔬菜生产商合作,进行该材料的生产与应用。
【总页数】1页(P40-40)
【关键词】生物降解;纳米微粒;Technologies公司;多层;塑膜;制成;德国;原料;生产企业;BIOP
【正文语种】中文
【中图分类】TU362;X172
【相关文献】
1.室温下具有1000Oe剩磁的磁纳米微粒研制成功 [J],
2.浸有纳米微粒的电子墨汁可制成传感器 [J], 杨英惠(摘译)
3.新PLA生物降解纳米材料研制成功 [J],
4.德国研制成无粘接多层阻隔膜 [J],
5.可生物降解高分子纳米微粒的制备及表征 [J], 葛海雄;蒋锡群;杨时成;杨昌正因版权原因,仅展示原文概要,查看原文内容请购买。
- 1、下载文档前请自行甄别文档内容的完整性,平台不提供额外的编辑、内容补充、找答案等附加服务。
- 2、"仅部分预览"的文档,不可在线预览部分如存在完整性等问题,可反馈申请退款(可完整预览的文档不适用该条件!)。
- 3、如文档侵犯您的权益,请联系客服反馈,我们会尽快为您处理(人工客服工作时间:9:00-18:30)。
J O U R N A L O F M A T E R I A L S S C I E N C E(2005)MuCell R technology for injection molding:A processing method for polyether-urethane scaffoldsS.LEICHER,J.WILL,H.HAUGEN,E.WINTERMANTELCentral Institute for Medical Engineering ZIMT,Technische Universit¨at M¨unchen, Boltzmannstrasse11,D-85748,Garching bei M¨unchen,GermanyMuCell R injection molding technology which takes supercritical carbon dioxide as blowing agent was used as processing method to generate porous samples made of thermoplastic polyether-urethane.The influence of processing parameters on the morphology of the porous structures was examined by mercury intrusion porometry and image analysis.An increase in injection speed and content of CO2in the polymer melt decreased pore sizes whereas an increase in the percentage of weight reduction increased pore sizes.Polyether-urethane samples with porosities of70%and pore sizes from184to1102µm were observed.Interconnective pore sizes ranged from40to275µm.C 2005Springer Science+Business Media,Inc.1.IntroductionPresent approaches for fabricating porous polymer structures for biomedical applications include partic-ulate leaching methods[1–3],phase separation[4], emulsion freeze-drying[5],and combinations of these. The named techniques use organic solvents which may be left in the scaffold and might cause inflammatory reactions[6].Newer approaches like carbon dioxide expansion avoid these solvents[6].Nevertheless,all afore mentioned methods are limited in number and size of the samples due to laboratory handling.No ideal scaffold processing technique suited for industrial pro-duction of porous scaffolds is yet known.Haugen[7,8]developed a polymer foaming method for injection molding which uses water as non toxic blowing agent combined with a salt leaching technique. Although this method is simple and is able to produce scaffolds with high porosities and pore sizes in the de-sired range,the major drawback of the process is the time-consuming preparation of the polymer and the necessary leaching step.Also,the hydrolysis of the chosen thermoplastic polyether-urethane at high tem-peratures in the presence of water[9]is a disadvantage. In this study,the MuCell R injection molding tech-nology which uses benign gas as blowing agent was observed concerning its ability to work as a large scale scaffold processing method for biomedical engineer-ing.The parameters of the process and their influ-ence on pore morphology were examined.As there are a large number of dependent process parameters,it was necessary to focus on key parameters.Fixed pro-cess parameters were the cooling time,plasticize pres-sure and microcellular process pressure(MPP),tem-perature of the heating bands and plasticizing rotation whereas the changeable parameters were CO2weight gain,percentage of weight reduction,injection speed, and temperature of the mold.The choice of the change-able parameters was done based on the knowledge given by nucleation theory and literature data[7,10]. Rodeheaver and Colton[11]found that an increased foaming temperature is an important factor to achieve a high content of open pores.Therefore,the highest possible temperature for the chosen material was set on the heating bands.Samples were produced by vary-ing one parameter while the others were held constant. Thus,the influence of each processing parameter on the pore morphology could easily be observed.After pro-cessing,the samples were subsequently analyzed using mercury intrusion porometry,optical analysis and me-chanical tests.2.Materials and methods2.1.Polymer processingThermoplastic polyether-urethane(TPU)(Texin R 985,Bayer Plastics Division,Pittsburgh,PA,USA) was chosen to process the samples.An injection mold-ing machine(KM125C2,Krauss Maffei GmbH, Munich,Germany)with a mold temperature control-ling device(90S,Regloplas GmbH,Munich,Germany) was used for the mass production of the porous sam-ples.The injection molding machine was equipped with a MuCell R package by the manufacturer.This package contains a special plasticizing unit(Sp220MuCell R plasticizing unit with a screw diameter of25.0mm and an adaptor for Sp520plasticize unit,Krauss Maf-fei GmbH,Munich,Germany)with one supercriti-calfluid(SCF)injection valve(25mm series II in-jector,Trexel Inc.,Woburn,MA,USA).It also in-cludes a SCF metering system that consists of a SCF0022-2461C 2005Springer Science+Business Media,Inc. DOI:10.1007/s10853-005-0853-yT A B L E I Variable andfixed parameters while processing Variable parameters Examined range CO2weight gain 1.6–6.3wt% Percentage of weight reduction(compared to bulk part)25–59% Injection speed30–150mm/s Fixed parameters ValueCooling time65sInjection pressure1500bar Plasticize pressure200barMPP200barDwell pressure200bar Beginning dwell pressure5mmDuration of dwell pressure0.5sClamp tonnage200kN Temperature of the heating bands180–210◦C Temperature of the mold40◦C Plasticizing rotation60min−1delivery system(TE-3series II,Trexel Inc.,Woburn, MA,USA)and piping as well as instrumentation.The used mold was capable to produce six toroid-shaped samples with an outer diameter of31mm and a thick-ness of10mm at one shot.Table I gives an overview over thefixed parameters and shows the analyzed range of the variable parameters that were used during the experiments.2.2.PorometryMercury intrusion porometry(AutoPore IV9500,Mi-cromeritics Instrument Corporation,Norcross,GA, USA)was applied to determine pore size distribution and porosity of the samples.The porous polymer sam-ples were placed in a solid penetrometer with5ml bulb volume(Solid5cc,Pen Stem.38cc,Micromerit-ics Instrument Corporation,Norcross,GA,USA).The intrusion chamber was thenfilled with mercury and the T A B L E I I Processing conditions to produce the samplesCO2contentPercentageof weightreductionInjectionspeed Varying the injection speed 1.8%25%–Varying the percentage ofweight reduction2.5%–150mm/s Varying the content of CO2–57%150mm/s Overall porosity 6.3%59%150mm/ssamples were penetrated with mercury until a maxi-mum pressure of110MPa,where the total intrusion volume reached a plateau.A curve resulting from a measurement consists of120measure points,the lines in Fig.1are drawn to guide the eyes only.The peak of a curve gives the mean pore size diameter.Mercury in-trusion measurements were done at samples produced with an injection speed of30and150mm/s,a per-centage of weight reduction of27and59%,and a con-tent of carbon dioxide of1.6respectively6.3%.The processing conditions that were used to produce the samples for mercury intrusion porometry are shown in Table II.2.3.Image analysisScanning electron microscope(SEM)(S-3500N, Hitachi Science Systems,Tokyo,Japan)was used for observation of the internal pore morphology.The porous samples were sliced with a scalpel and then coated with gold by using a sputter-coater(SCD005, BAL-TEC AG,Balzers,Lichtenstein).The morphol-ogy of the pores and the connections was evaluated by using image-processing analysis(Cellenger4.0, Definiens AG,Munich,Germany)based on SEM mi-crographs.The rule sets were created especially for this application and are explained in the following.The area of an image object is the number of pixels formingit. Figure1Pore size distribution as a function of the injection speed(top),the percentage of weight reduction and the content of carbon dioxide (bottom).T A B L E I I I Parameters for the quasi-static tensile-test Parameters ValueAmbient medium Air,room temperature Initial load5NInitial load speed50mm/minTest speed500mm/minLoad cell 2.5kNDerived from the object area is the pore diameter which gives the diameter of a round pore with the same area. The average diameter of all pores that can be found in one image is the mean pore diameter.In the follow-ing this value is referred to as median pore diameter and describes the size of the interconnections of adja-cent voids.Due to the fact that the pores shown in the micrographs are two-dimensional projections of three-dimensional objects,their maximum diameter may not be represented in the image.To consider this,a factor of0.616was used to determine the maximum spherical diameter,called corrected median pore diameter,from the measured median pore diameter.Image analysis was done at samples produced with an injection speed of30to150mm/s in increments of30mm/s,a percent-age of weight reduction of27and59%,and a content of carbon dioxide of1.6respectively6.3wt%.Table II gives the processing conditions that were used to pro-duce the samples for mercury intrusion porometry and image analysis.2.4.Mechanical analysisRing shaped samples went through a quasi-static tensile-test with a material testing machine(TC-FR 2.5TS.D09,Zwick GmbH&Co.,Ulm,Germany) under conditions defined by DIN53504.Table III lists the chosen experimental parameters.The tested samples were processed with an injection speed of 150mm/s,a gas content of2.15wt%(±0.35%)and a percentage of weight reduction of25respectively 59%.3.Results3.1.PorometryFig.1shows the influence of the injection speed,the percentage of weight reduction,and the content of car-T A B L E I V Average porosity and Standard deviation.A molded part consists of six toroid-shaped samples,the sprue and the runner system Tested samples Average porosity One toroid(n=7)70.4±3.8% One moulded part(n=6)69.6±2.9% Series of moulded parts(n=6)69.5±2.7% Overall porosity(n=19)69.9±3.2%bon dioxide on pore morphology,measured by mercury intrusion porometry.At a speed of30mm/s the mean pore size diameter(top section of Fig.1)was at300µm with a porosity of77%.An increase of the injec-tion speed to150mm/s shifted the mean pore size to45µm with a porosity of72%.The pore size distribution of the samples processed with150mm/s was narrower than the distribution obtained at the injection speed of 30mm/s.The mean pore size diameter at59%weight reduction(middle section of Fig.1)was at345µm with a porosity of68%.At a percentage of weight reduction of27%the mean pore size was at60µm with a poros-ity of66%.At59%weight reduction a very broad pore distribution with several peaks of mercury intrusion was visible,whereas at the lower percentage of weight reduction the pore size distribution was narrower.The most common pore size for samples processed with 1.6wt%carbon dioxide(bottom section of Fig.1)was 165µm with a porosity of74%.A gas content increase to6.3wt%shifted the mean pore size diameter to12µm with a porosity of75%.The pore size distribution of both curves was of nearly similar shape.Table IV displays the average porosities and standard deviations of the tested samples.The porosity of one molded part was70.4±3.8%.Samples taken from six different toroids of one shot showed an average porosity of69.6±2.9%.The porosity of samples taken from a series of molded parts was69.5±2.7%.This leaded to an overall porosity of69.9±3.2%.3.2.Image analysisTypical SEM micrographs of samples processed with different injection speeds can be seen in Fig.2.The pictures display that pore size decreased with an in-crease in injection speed from30(left)to150mm/s. Further on an orientation of the pores depending on theflow path of the polymer melt was apparent.The morphology of the pores at different percentagesof Figure2Pore morphology at injection speeds of30(left)respectively150mm/s.Figure 3Pore morphology at 27(left )and 59%of weightreduction.Figure 4Pore morphology at 1.6(left )and 6.3wt%of gas content.T A B L E V Diameters of the connections between neighboring pores in dependence on the variable parameters Parameter Value Median pore diameter Injection speed 30mm/s 275±89µm 150mm/s 53±27µm Percentage of weight reduction 27%40±15µm 59%59±25µm Content of CO 21.6wt%85±45µm 6.3wt%50±19µmweight reduction is shown in Fig.3.The most obvious difference between the pictures was that at 27%weight reduction (left)the pores had a uniform structure.On the other hand at higher weight reduction (59%)the pores differ much in size.Samples processed with dif-ferent gas contents are visible in Fig.4.The structure with the gas content of 6.3wt%,shown on the right side,contained more small pores and also seemed to be less interconnective.Fig.5and Table V present the computational analysis of the SEM micrographs.Fig.5describes the influence of the injection speed,the percentage of weight reduc-tion,and the content of carbon dioxide on the corrected median pore diameter.An increase in injection speed decreased pore sizes.At an injection speed of 150mm/s the most uniform structure with median pore sizes of 284±29µm could be found.An injection speed of 30mm/s generated pores with a median diameterof 1102±406µm.At a percentage of weight reduc-tion of 27%the median pore diameter could be found at 184±37µm.A percentage of weight reduction of 59%generated pores with a median diameter of 370±287µm.At a gas content of 1.6wt%the median pore diameter was at 571±354µm.6.3wt%carbon dioxide in the polymer melt leaded to pores with a pore diameter of 307±214µm.Table V shows the diameters of the connections be-tween neighboring pores in dependence on the varied parameters.At an injection speed of 30mm/s the pore diameter was 275±89µm.An increase in injection speed to 150mm/s leaded to a connection size of 53±27µm.A variation in the percentage of weight reduc-tion and the content of CO 2produced openings with a size of 40±15µm at 27%and 59±25µm at 59%of weight reduction respectively 85±45µm at 1.6wt%and 50±19µm at 6.3wt%CO 2.3.3.Mechanical propertiesFig.6shows the mechanical properties of the molded samples.The breaking force for the specimen pro-cessed with 25%weight reduction (n =20)was 623±124N.The elongation at break could be found at 190±39%.Compared to that a percentage of weight reduction of 59%(n =20)leaded to a breaking force of 249±105N and an elongation at break of 76±41%.Figure5Corrected median pore diameter in dependence on the injection speed,the percentage of weight reduction,and the gascontent.Figure6Mechanical properties of the samples in dependence on the percentage of weight reduction.4.ConclusionIt was observed that the pore morphology depended on several adjustable processing parameters.The pore size and the pore size distribution were adjustable by the injection speed,the percentage of weight reduc-tion and the content of carbon dioxide in the polymer melt.The porosity of the samples was not much in-fluenced by these parameters.The injection speed that generates the rate of the pressure drop which is a main factor to generate nucleation sites[12,13],had a ma-jor effect on pore size and pore size distribution.An increase in injection speed decreased the pore sizes and caused a narrower pore size distribution.When comparing the results of the pore sizes obtained from mercury intrusion porometry and image analysis it can be seen that the pores measured by porometry are much smaller than those measured by image analysis.This was likely caused by the so-called inkstand effect.This means that a small pore with a large void behind ap-pears as many small pores in the pore size distribution curve.The result showed that an increase in injection speed from30to150mm/s decreased the corrected median pore diameter from1102to284µm.The anisotropic orientation of the pores comes about be-cause the mechanical stresses generated during foam-ing are not distributed uniformly through the volumeof the polymer foam.Therefore the gas bubbles tend to expand along the directions of minimum local stress [14].At a percentage of weight reduction of27%the cor-rected median pore diameter was found to be184µm.A degree of weight reduction of59%generated pores with a median diameter of370µm.Lower degrees of weight reduction caused structures with a narrow pore size distribution.A high degree of weight reduction leaded to a non uniform cell structure.This effect is verified by literature data[15].At lower weight reduc-tion the skin of the part was much thicker(results not shown)and the parts were much harder to remove from the mold.An influence of the percentage of weight re-duction on the degree of interconnection was not found whereas the size of the connections at a high percentage of weight reduction was larger with a larger standard deviation.A percentage of weight reduction up to59% was achieved.Further weight reduction was not possi-ble since the molded part could not be removed from the mold.The percentage of weight reduction had a ma-jor effect on the mechanical properties of the porous samples.The breaking force and also the elongation at break for the specimen processed with25%weight reduction are2.5times higher than those obtained at a percentage of weight reduction of59%.Since there was no significant influence of the weight reduction on porosity this effect must result from the thicker skin of the samples processed with the lower degree of weight reduction.At a gas content of1.6wt%the mean pore size diameter was at170µm whereas a gas content of 6.3wt%shifted the mean pore size to12µm.It is known that a high gas content leads to more nucleation sites and thus smaller pores[15].The results obtained from mercury intrusion measurements differed much from those obtained from image analysis.The samples were processed with a high percentage of weight reduc-tion which caused a very broad pore size distribution. As image analysis only exploits a small detail of the porous structure a few large pores among many small pores will increase the median pore diameter and also leads to a huge standard deviation.The reproducibility of the processing method con-cerning the porosity was proven.Overall,samples with a median porosity of69.9±3.2%(n=19)were pro-duced.In this study it has been shown that MuCell R tech-nology for injection molding is a useful processing method to generate porous cell carriers made of ther-moplastic polyurethane in a large scale.The processed samples show an open porous structure which was achieved without the use of additives such as NaCl. References1.A.G.M I KO S,A.J.T H O R S E N,L.A.C Z E RW O N K A,Y.BAO,R.L A N G E R,D.N.W I N S L OW and J.P.VA-C A N T I,Polymer35(1994)1068.2.D.J.M O O N E Y,S.PA R K,P.M.K AU F M A N N,K.S A N O,K.M C NA M A R A,J.P.VAC A N T I and R.L A N G E R,J.Biomed.Mater.Res.29(1995)959.3.R.C.T H O M S O N,M.J.YA S Z E M S K I and J.M.P OW-E R S,J.Biomater.Sci.Polym.Ed.7(1995)23.4.H.L O,M.S.P O N T I C I E L L O and K.W.L E O N G,Tissue.Eng.(1995)15.5.K.W H A N G,T.K.G O L D S T I C K and K.E.H E A LY,Bio-materials21(2000)2545.6.D.J.M O O N E Y,D.F.BA L DW I N,N.P.S U H,J.P.VAC A N T I and R.L A N G E R,ibid.17(1996)1417.7.H.J.H AU G E N,in“Development of an Implant to Treat Gastro-Oesophageal Reflux Diseases”(Ph.D.Thesis,Technical University Munich,2004).8.H.H AU G E N,V.R I E D,M.B RU N N E R,J.W I L L and E.W I N T E R M A N T E L,J.Mater.Sci.Mater.M.15(2004)343.9.N.M.K.L A M BA,K.A.W O O D H O U S E and S.L.C O O P E R,in“Polyurethanes in Biomedical Applications”(CRCPress,Boca Raton,1998)p.181.10.H.K AWA S H I M A and M.S H I M B O,Cell.Polymers22(2003)175.11.B.A.RO D E H E AV E R and J.S.C O LTO N,Polym.Eng.Sci.41(2001)380.12.C.B.PA R K,D.F.BA L DW I N and N.P.S U H,ibid.35(1995)432.13.V.G O O D S H I P,R.L.S T E WA R D,R.H A N S E L L,E.O.O G U R and G.F.S M I T H,Cell.Polymers23(2004)25.14.F.A.S H U TOV,in“Handbook of Polymeric Foams and FoamTechnology”(Hanser,Munich,1991)p.30.15.K.T.O K A M OTO,in“Microcellular Processing”(Carl Hanser,Munich,2003)p.177.Received10December2004and accepted7March2005。